Part of a series of articles titled Alaska Park Science, Volume 18, Issue 1, Understanding and Preparing for Alaska's Geohazards.
Article
The 2015 Taan Fiord Landslide and Tsunami
Marten Geertsema, University of Northern British Columbia
Dan Shugar, University of Calgary
Patrick Lynett, University of Southern California
Anja Dufresne, Rheinisch-Westfälische Technische Hochschule Aachen University, Germany
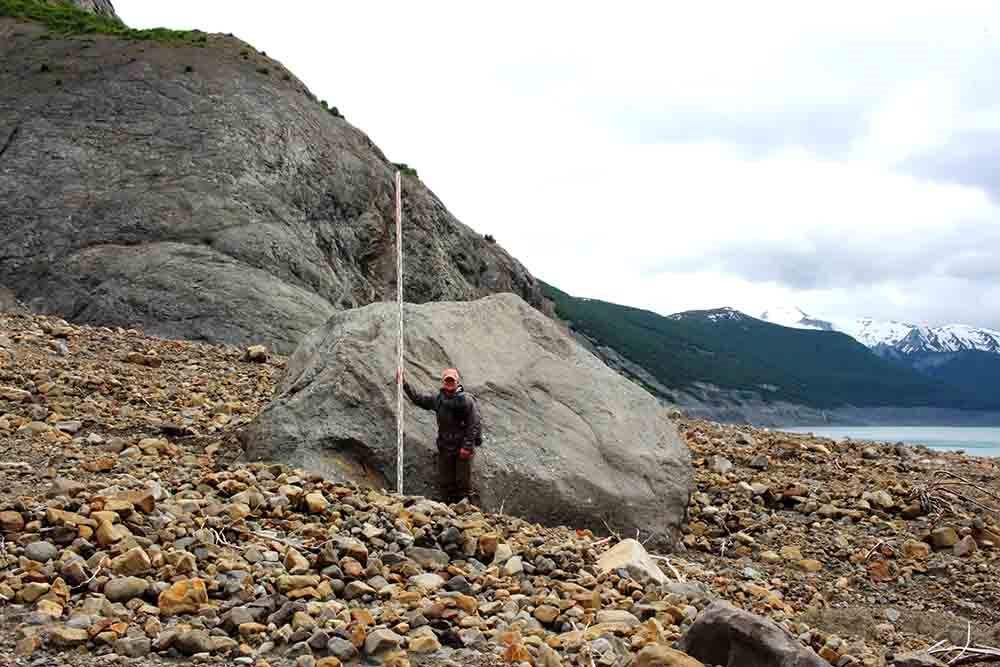
Photo courtesy of Ground Truth Trekking
On October 17, 2015, 180 million tons of rock slid into Taan Fiord, an arm of Icy Bay, generating a tsunami that stripped forest from 8 square miles (20 square km) of Wrangell St.-Elias National Park and Preserve and reached as high as 633 feet (193 m) above the fjord, the fourth-highest tsunami ever recorded. It had almost no human impacts—nobody was near enough to be harmed, and the only damage to infrastructure was rocks scattered on a beach used for landing bush planes—good fortune that may not characterize similar events in the future.
Alaska parks are prone to events like this. Land-slides that generate large tsunamis most often happen in landscapes with retreating glaciers. In the last century, 10 of the 14 highest tsunamis in the world were in glaciated mountains and four were in Alaska parks, which include vast tracts of glaciated terrain (Table 1). Though landslides like this can happen at any time, these events are becoming more frequent—driven by climate change-induced glacial retreat and permafrost thaw. The Taan Fiord tsunami can help us understand subaerial landslide tsunami hazards and prepare for potential impacts.
Year | Location | Water Body | Cause | Latitude | Longitude | Max Runup (m) |
1958 |
Lituya Bay, Alaska, USA |
Fjord |
Subaerial landslide |
58.672 |
-137.526 |
524 |
1980 |
Spirit Lake, Washington, USA |
Lake |
Volcanic landslide |
46.273 |
-122.135 |
250 |
1963 |
Casso, Italy |
Reservoir |
Subaerial landslide |
46.272 |
12.331 |
235 |
2015 |
Taan Fiord, Alaska, USA |
Fjord |
Subaerial landslide |
60.2 |
-141.1 |
193 |
1936 |
Lituya Bay, Alaska, USA |
Fjord |
Subaerial landslide |
58.64 |
-137.57 |
149 |
2017 |
Nuugaatsiaq, Greenland |
Fjord |
Subaerial landslide |
71.8 |
-52.5 |
90 |
1936 |
Nesodden, Norway |
Fjord |
Subaerial landslide |
61.87 |
6.851 |
74 |
1964 |
Cliff Mine, Alaska, USA |
Fjord |
Delta-front failure |
61.125 |
-146.5 |
67 |
1934 |
Tafjord, Norway |
Fjord |
Subaerial landslide |
62.27 |
7.39 |
62 |
1965 |
Lago Cabrera, Chile |
Lake |
Subaerial landslide |
-41.8666 |
-72.4635 |
60 |
1967 |
Grewingk Lake, Alaska, USA |
Lake |
Subaerial landslide |
59.6 |
-151.1 |
60 |
1946 |
Mt. Colonel Foster, British Columbia, Canada |
Lake |
Subaerial landslide |
49.758 |
-125.85 |
51 |
2004 |
Labuhan, Indonesia |
Open Coast |
Earthquake displacement |
5.429 |
95.234 |
51 |
2000 |
Paatuut, Greenland |
Fjord |
Subaerial landslide |
70.25 |
-52.75 |
50 |
Tsunamis from Landslides
Tsunamis are water waves generated by a sudden force (Bourgeois 2009), typically through either rapid displacement of the seafloor (during earthquakes or from submarine landslides), or through displacement and impact (by mountain landslides entering water, glacial ice collapses, or meteorite impacts). Depending on the geometry, size, and speed of this force, the nature of the resulting tsunami wave can vary dramatically. Tsunamis caused by earthquakes are typically long-period waves, meaning they rise and fall gradually. They can impact areas as large as entire ocean basins, but with shorter runups (they run up from the coast to lower elevations), as happened with the Indian Ocean tsunami in 2004, and the Tohoku, Japan tsunami in 2011. These long-period tsunamis usually take up to a half-hour to inundate elevations less than twenty meters above the water. In contrast, tsunamis generated by subaerial landslides (as well as ice-calving and meteor impacts) are often much shorter-period, flooding the land more rapidly, and affect a smaller area. They crash ashore more like a wind wave, rising to their peak elevations within a few minutes at most. These tsunamis may reach several hundred meters above the water, but affect a much smaller area than their long-period cousins. Tsunamis generated by subaerial landslides are often confined to a few tens of kilometers around the landslide, both because shorter-period waves disperse energy more quickly and because they commonly are triggered in relatively confined bays and lakes.
Most of the research on tsunami impacts has focused on longer-period regional tsunamis—especially subduction zone earthquake tsunamis that produce some of the longest period waves. However, due to the differences outlined above, it is unclear how much of this work can be directly applied to tsunamis from subaerial landslides. The need to understand short-period landslide generated tsunamis was a major motivation for our study of the Taan Fiord event.
In Alaska, four giant tsunamis have been triggered by subaerial landslides in the past century: in Lituya Bay in 1938 and 1958 (Miller 1960); in Grewingk Lake in 1967 (Wiles and Calkin 1992); and in Taan Fiord in 2015 (Higman et al. 2018). Numerous landslides and at least two smaller landslide tsunamis have occurred in Alaska recent years (e.g., Geertsema 2012, Coe et al. 2018, Gullufsen 2018).
Climate warming is likely driving an increase in the frequency of large landslides and promoting the growth of deep bodies of water where large tsunamis can form. Glaciers simultaneously erode the base of mountain slopes, impose stresses, and induce fracturing, all of which increase the likelihood of slope failure. At the same time, glaciers fill the valley, preventing failure of lower slopes and supporting higher slopes to reduce the chance of slope collapse. When they retreat, whether due to changing climate or to regular glacial cycles, they remove that support, allowing slopes to sag and fractures to expand, making them vulnerable to failure. Furthermore, at higher elevations and in arctic and subarctic climates, permafrost is a significant contributor to the strength of some mountains, and thawing, or even warming of still-frozen permafrost, can greatly weaken those mountains. These factors are likely driving an increase in the frequency of large landslides. Additionally, glacial retreat is exposing new bodies of water that may be vulnerable to tsunamis. The events at Grewingk Lake, Taan Fiord, and a 2016 landslide tsunami in Cowee Creek near Juneau all occurred in bodies of water that didn’t exist (because their basins were filled with ice) only a few decades before.
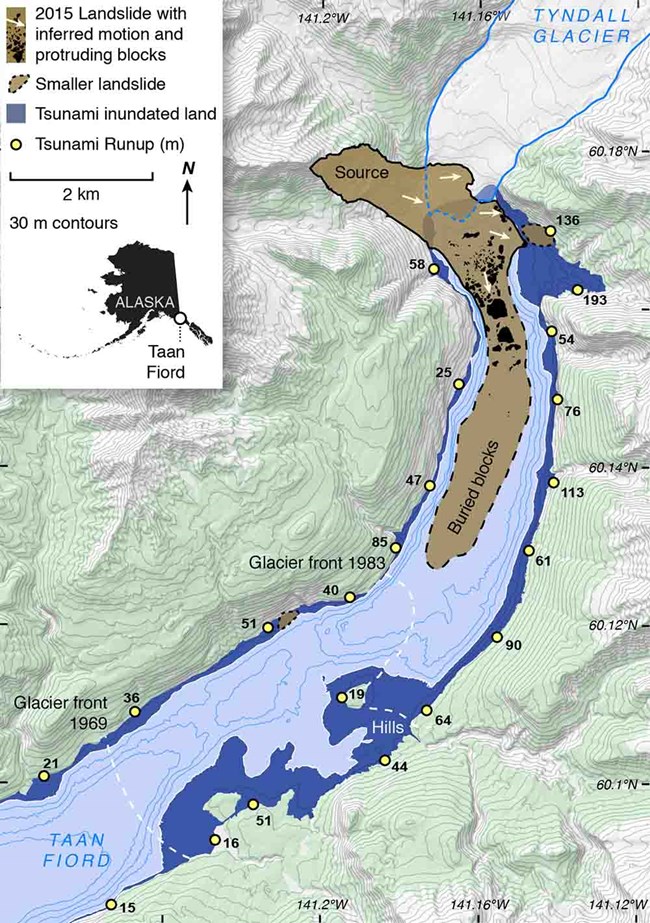
The Taan Fiord Landslide Tsunami
The Taan Fiord tsunami was preceded by a century of rapid glacial retreat (Koppes and Hallet 2006), decades of ground cracking and creep (Meigs et al. 2006), a month of above-average rain, and a few seconds of mild shaking from a distant earthquake (Higman et al. 2018). After crossing the toe of Tyndall Glacier and entering the water, it generated a violent wave that flattened about eight square miles (20 km2) of forest in Wrangell-St. Elias National Park and Preserve and adjacent private lands (Figure 1).
No one observed the slide or tsunami directly, but automatic seismic systems identified it within hours (using methods from Ekström and Stark 2013). Inversion of long-period waves arriving at nearby seismometers identified the general area and direction of the landslide. Days later, high-resolution satellite imagery revealed the landslide and tsunami impacts, and tide gage data from more than 90 miles (~150 km) away at Yakutat showed small fluctuations in water level (4 inches or ~0.1 m) resulting from the tsunami. Months later, teams of scientists visited the site to document both the landslide and tsunami.
The landslide began as a rotational slide, likely following planes of weakness established decades earlier when failure initiated shortly after glacial retreat. Signs of active displacement are clearly visible in remote-sensed data extending back to the mid-1990s. A decade before catastrophic failure in 2015, Meigs and others (2006) noted ground-cracking and deformation, and aerial topographic surveys documented tens of meters of gradual motion (Higman et al. 2018).
After the failure in 2015, a portion of the slide block remained on the slope, still covered by jumbled, but still largely upright alder forest (Dufresne et al. 2018). However, most of the slide mass sped across the shoreline and terminus of Tyndall Glacier and entered Taan Fiord. Multibeam sonar and sub-bottom profiling show blocky deposits capped by more homogenous layered deposits filling the fjord bottom (Haeussler et al. 2018). A small portion of the landslide traveled across the fjord bottom and slid back up above the waterline on the far side of the fjord, forming 30-70 feet-high (10-20 m) debris hillocks. Several alluvial fans collapsed into deeper water as the slide moved along their fronts on its way down the fjord. These collapsed fans left no clear deposit—presumably because they were incorporated into the moving submarine slide mass.
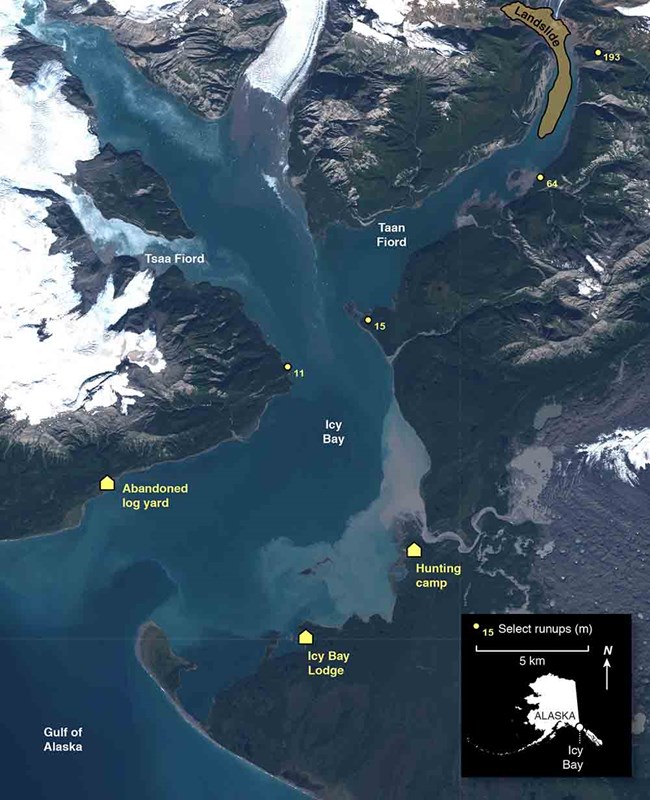
The impact of the slide with the water generated one of the tallest tsunami waves in historical times. At one location, the wave reached 633 feet (193 m) above sea level. As it moved down the fjord, the wave stripped vegetation to varying heights that gradually diminished down-fjord. At the mouth of the fjord, 10 miles (16 km) from the landslide, the wave reached 50 feet (15 m), and dragged icebergs through moraine hills. Two-and-one-half-miles (4 km) farther, on a section of the west coast of Icy Bay that faces directly toward the mouth of Taan Fiord, the wave reached 36 feet (11 m) and toppled trees, but quickly diminished to below high tide as it spread out along the coast (Figure 2). The faint echo of the tsunami reached tide gages 80 and 250 miles (130 and 400 km) away where they recorded 40-minute-long oscillations of several inches in water level.
Potential for Future Disaster in Icy Bay
The Taan Fiord event provides warning that Icy Bay may see similar, potentially more deadly events in the future. Lituya Bay produced at least five giant tsunamis over the course of three centuries (Miller 1960) and Icy Bay could well rack up a similar record.
A century ago, Icy Bay was filled with glaciers (Russel 1893). It wasn’t until the 1960s that four steep-walled fjords began to open at the head of the bay. The 2015 tsunami was the largest to occur in this narrow window of time, but it likely wasn’t the only tsunami. At least two sites (see Figure 1) along the fjord have fresh landslide deposits extending into the ocean, and a ridge opposite the 2015 slide is laced with fissures that may produce future failures. Other fjords at the head of Icy Bay have steep slopes that haven’t yet been surveyed for potential landslide hazards.
Adventure kayakers, trophy bear hunters, commercial and sport fishers, and even cruise ships visit Icy Bay (Figure 2). Though logging along its shore has ended, plans for a large-scale mine are being explored, potentially creating another vulnerable facility. When the tsunami occurred in October 2015, workers were present in Icy Bay Lodge just 20 miles (32 km) away, fortunately beyond damaging waves. However, many similar situations might have had greater impact: A landslide might occur at a busy time of year; a small landslide or tsunami might impact a beach campsite; or a large landslide might occur in one of the other fjord arms of Icy Bay. Due to the geometry of the bay, a tsunami in a different arm would more directly impact the outer part of Icy Bay, where there is more human activity.
How an Event like the 2015 Landslide and Tsunami Might Play Out Elsewhere
Landslide Impacts
In addition to the complete destruction in the path of the slide, the 2015 Taan Fiord landslide reshaped the fjord bottom and far shoreline. The slide caused tens of meters of shallowing in some areas, while it eroded sections of the pre-landslide shoreline. Changes like this can render existing nautical charts unreliable and might mean that they need to be updated with new bathymetric surveys before large ships can return to waters impacted by similarly large landslides.
Tsunami Hazards to Vessels
The most dramatic hazard posed to vessels is the breaking tsunami wave in areas where the tsunami height is significant in comparison to the depth of the water. Runup of nearly 650 feet (200 m) shows that the Taan Fiord tsunami was likely about 300 feet (100 m) in height, and complex variability in the peak runup shows the wave had a very short period. A wave of such extreme amplitude and short wavelength would be likely to break even at fjord depths of hundreds of feet. Like all tsunami waves, it would also be prone to breaking in shallow water.
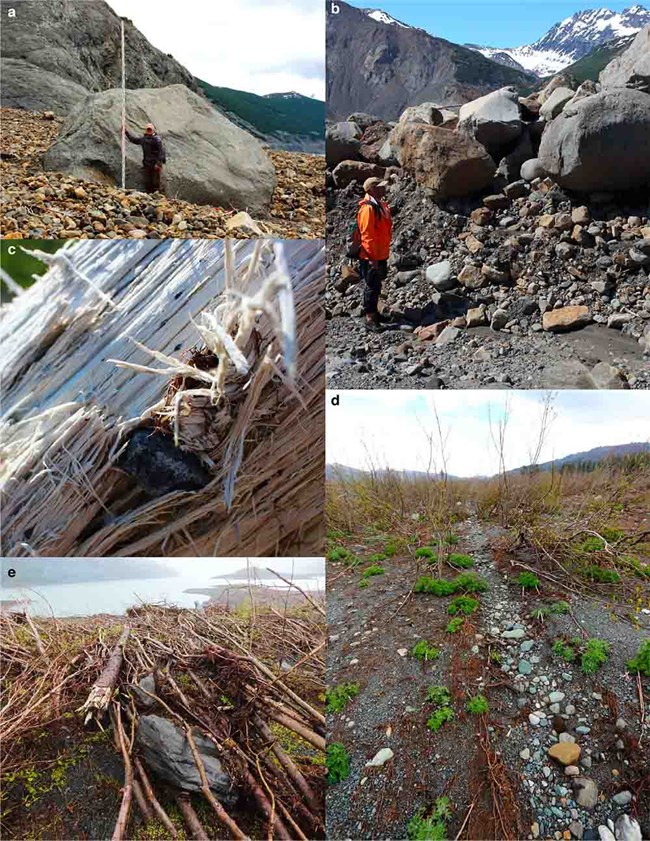
Even where the wave is not breaking, tsunamis can generate strong currents. In Taan Fiord, currents swept across shallow areas and low hills, sometimes carrying icebergs that left gouges in the soil surface (Figure 3), or were left stranded far above the tide. Ships carried in such currents would be vulnerable to collisions that could rupture the hull, or they might be carried broadside into shoals where they could be rolled over. Smaller vessels might experience some of the same hazards as can be found in tidal rapids—strong eddy-lines and whirlpools that can capsize and sink boats. Finally, in troughs between waves vessels might ground on obstacles that would otherwise pose no threat.
It is possible to anticipate areas of potential hazard for vessels using tsunami models. One rough result has been published (not related to our study) that jointly models the landslide and tsunami (George et al. 2017). We are currently working to model the tsunami in more detail, using a numerical model tested against tectonic tsunamis. However, modeling to simulate possible future events will be very sensitive to potential landslide locations, requiring many model runs to capture a range of possible scenarios. Also, while modeled tsunami runup has been widely validated, currents produced by tsunami flows are rarely directly documented, and tsunami models differ substantially in the results they produce (Lynett et al. 2012). Current velocity validation using natural indicators of previous tsunami flow, or using direct observations of tsunamis, are badly needed.
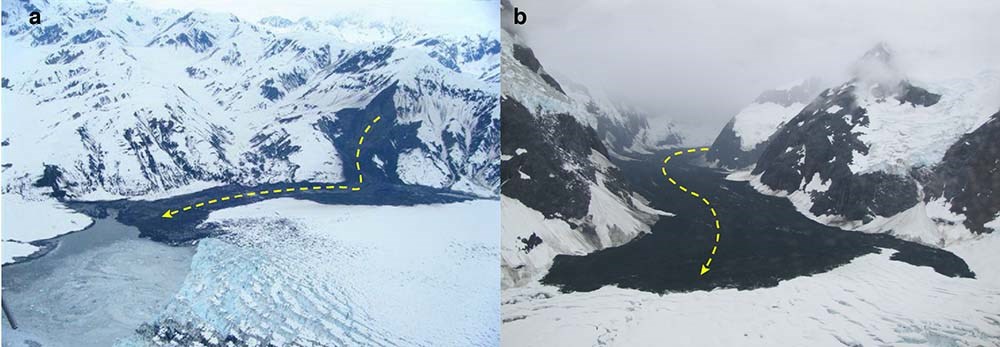
The larger Lituya landslide (b) came to rest more than 6 miles (10 km) from tidewater in Glacier Bay National Park and Preserve, and thus produced no tsunami.
Not all Alaskan rockslides possess the same potential as the Taan Fiord event for generating giant waves that might endanger vessels. While most of the Taan landslide debris entered the fjord, in other cases, the percentages of debris deposited in water bodies may be minimal to non-existent. Figure 4 shows the 2012 Hubbard and Lituya landslides (Geertsema 2012). Even though some of the Hubbard landslide entered Disenchantment Bay, it was only a small proportion of the distal landslide deposit—thus the wave was very small—overtopping the beach by less than 6 feet (2 m). The Lituya slide, though very large, came to rest some 6 miles (10 km) from tidewater. Nonetheless, the hazard from large landslide-generated waves will likely increase in the coming decades. Large landslides are on the increase in southeast Alaska (Coe et al. 2018), and as glaciers continue to migrate landwards, water bodies such as fjords and lakes will also grow landward toward steep, recently deglaciated terrain, increasing the potential for more landslides, more “direct hits” into water, and therefore more, and possibly larger landslide-generated tsunamis.
- Duration:
- 1 minute, 24 seconds
VIDEO: Camp Bay, velocity. Numerical simulation of the Taan Fiord tsunami, here showing the speed of the moving water. The colors on the tsunami indicate the speed, with the reds indicating tsunami flow speeds of 30 m/s (~65 mph). The black arrows show only the direction of the moving water; together with the color the viewer is able to see both the magnitude and direction of the tsunami flow. The location shown here is near the mid-length of Taan Fiord, where the survey team set up their primary base camp. For the readers reference, the force exerted on a object submerged in water moving at 65 mph is the same as the force felt by that object with wind blowing at 2,000 mph.
- Duration:
- 29 seconds
VIDEO: Camp Bay, height Numerical simulation of the Taan Fiord tsunami, showing the height of the moving water above the tide level. The colors on the tsunami indicate the height, with the reds indicating tsunami crest elevations of 40 m (130 ft) above the tide and the dark blues showing tsunami trough depressions of 20 m (65 ft) below the tide level. The location shown here is near the mid-length of Taan Fiord, where the survey team set up their primary base camp. Note how the tsunami is strongly influenced by the topography, with large changes in run-up elevations over short distances.
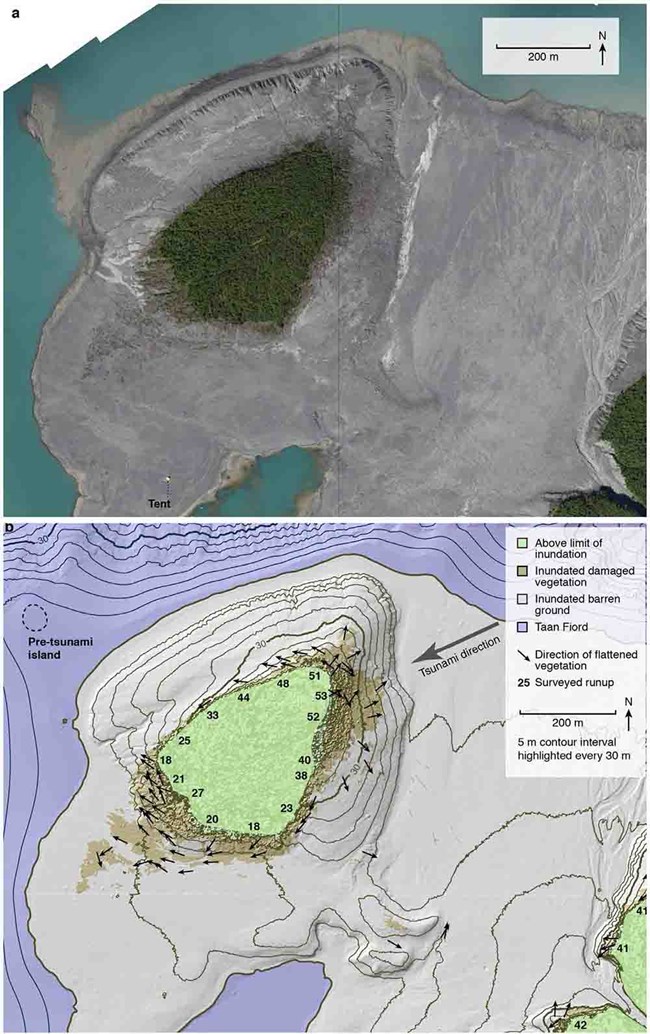
Onland Tsunami Hazards
Deforestation of the slopes above Taan Fiord demonstrates the destructive power of the tsunami (Figure 3). In most places, the forest was destroyed by the passing wave. The degree of destruction increased away from the limit of inundation, areas 60 feet (20 m) below that limit were typically so hard-hit that only a few torn roots and soil remnants remained, while trees near the limit were often toppled but still rooted. Some forest remained standing along steep slopes with runups of about 30 feet (10 m), and patches of dense spruce forest at the mouth of the fjord survived where rafts of debris formed dams to protect them from the iceberg-laden flow.
The tsunami moved boulders up to 16 feet (5 m) in diameter where it was largest (Figure 3). In some areas where there was abundant loose sediment, deposits of everything from boulders to sand were so thick that they raised ground elevations by over 16 feet (5 m). Deposits were more typically 12-20 inches (30-50 cm) thick over areas as far as 6 miles (10 km) from the source. In contrast, one small island that used to support supra-tidal vegetation was scoured down such that now it is only a shoal emerging at low tide (location marked on Figure 5). Tree trunks that remained standing after the tsunami were scoured by strong sediment-laden currents that sometimes severely abraded the upstream side of trees, leaving them peppered with small rocks (Figure 3). Assuming these trees were originally circular in cross-section, some must have lost at least 4 inches (10 cm) of wood to achieve the scoured shape we observed.
A few sites showed that hills can provide significant protection from the worst tsunami impacts. An 800 foot (250 m) diameter hill that was surrounded but not overtopped by the tsunami had runups of over 160 feet (50 m) where the tsunami directly impacted it, but less than 65 feet (20 m) on the lee side (Figure 5). Even below the inundation line on the protected side of this hill, there were more rooted trees and intact soil than in less-protected areas. A structure built here or in analogous locations would likely fare better even than more exposed locations that are significantly farther from the tsunami source.
Where there was little loose sediment, the tsunami typically scoured the land down to bedrock, and left little in the way of deposits. Presumably what little sediment was available in these areas was transported offshore.
These impacts in Taan Fiord show that development should be sited outside areas of anticipated inundation, and evacuation routes are needed so that anyone within the flood zone can effectively flee. Additionally, some consideration should be given to sediment transport: rip rap boulders could be carried by a tsunami, increasing damage and recovery costs. Areas near stream deltas or other sediment sources might be buried by transported sediment. Ideally, new development in areas of potential tsunami inundation could be designed to maximize options for evacuation and minimize costs of reconstruction after an event.
Anticipating Future Events
Alaska’s parks could do a lot to be better prepared for future events like this, whether in Icy Bay or anywhere else where steep glaciated mountains rise above deep water. Systematic field surveys of potential sources and remote analysis and monitoring of these sources for precursory movement could help identify likely landslide sites. Modeling based on potential sources can help outline areas of likely impact, with an emphasis on sites with known risk factors. These results could then be compared to areas of infrastructure development, popular tourist destinations, and ship course data (e.g., from Automatic Identification System (AIS) ship-tracking systems) to identify areas of particular vulnerability. A systematic analysis of this sort could inform park development planning and public education efforts that could reduce risk.
The Taan Fiord event could have been anticipated based on decades of precursory movement that preceded catastrophic failure, had such analysis been conducted prior to the failure. Acceleration revealed by active monitoring such as continuous GPS stations on the slide mass or satellite radar interferometry analysis can provide forewarning of imminent failure. The Taan Fiord event would have been a good candidate for active monitoring because precursory motion generated large, easily measured displacements (e.g., visible even in low-resolution Landsat imagery). Lodge operators and visitors might have been warned of the risk; tsunami modeling likely could have accurately shown the impacts would be potentially severe within the fjord, but would only extend a short way into the main bay. However, no such preparation was conducted and it’s urgent that we learn from the 2015 event and take steps to assess and mitigate hazards in Icy Bay and elsewhere before another event happens.
References
Bourgeois, J. 2009.
Geologic effects and records of tsunamis. The Sea 15: 53–91.
Coe, J. A., E. K. Bessette-Kirton, and M. Geertsema. 2018.
Increasing rock-avalanche size and mobility in Glacier Bay National Park and Preserve Alaska detected from 1984 to 2016 Landsat imagery. Landslides doi:10.1007/s10346-017-0879-7
Dufresne, A., M. Geertsema, D. H. Shugar, M. Koppes, B. Higman, P. J. Haeussler, C. Stark et al. 2018.
Sedimentology and geomorphology of a large tsunamigenic landslide, Taan Fjord, Alaska. Journal of Sedimentology 364: 302-318.
Ekström, G. and C. P. Stark. 2013.
Simple scaling of catastrophic landslide dynamics. Science 339: 1416–1419.
Geertsema, M. 2012.
Initial observations of the 11 June 2012 rock/ice avalanche, Lituya mountain, Alaska. in The First Meeting of International Consortium of Landslides Cold Region Landslides Network, Harbin, China 49–54. https://doi.org/10.1016/j.sedgeo.2017.10.004
George, D. L., R. M. Iverson, and C. M. Cannon. 2017.
New methodology for computing tsunami generation by subaerial landslides: Application to the 2015 Tyndall Glacier landslide Alaska. Geophysical Research Letters 44: 7276–7284.
Gullufsen, K. 2018.
Scientists discover rare ‘alpine tsunami’ occurred after massive 2016 rockfall near Juneau, Juneau Empire 14 Feb 2018. Available at: https://www.juneauempire.com/news/scientists-discover-rare-alpine-tsunami-occurred-after-massive-2016-rockfall-near-juneau/ (accessed May 14, 2019)
Haeussler, P. J., S. P. S. Gulick, N. McCall, M. Walton, R. Reece, C. Larsen, D. H. Shugar, M. Geertsema, J. G. Venditti, and K. Labay. 2018.
Submarine deposition of a subaerial landslide in Taan Fjord, Alaska. Journal of Geophysical Research-Earth Surface 123(10): 2443-2463.
Higman, Bretwood, Dan H. Shugar, Colin P. Stark, Göran Ekström, Michele N. Koppes, Patrick Lynett, Anja Dufresne et al. 2018.
The 2015 landslide and tsunami in Taan Fjord, Alaska. Scientific Reports 8(1): 12993. doi:10.1038/s41598-018-30475-w
Koppes, M. and B. Hallet. 2006.
Erosion rates during rapid deglaciation in Icy Bay Alaska. Journal of Geophysical Research 111 no. F2. DOI:10.1029/2005JF000349.
Lynett, P., J. Borrero, R. Weiss, S. Son, D. Greer, and W. Renteria. 2012.
Observations and modeling of tsunami-induced currents in ports and harbors. Earth and Planetary Science Letters 327-328:68-74.
Meigs, A., W. C. Krugh, K. Davis, and G. Bank. 2006.
Ultra-rapid landscape response and sediment yield following glacier retreat Icy Bay, southern Alaska. Geomorphology 78: 207–221.
Miller, D. J. 1960.
Giant waves in lituya bay, Alaska. (US Government Printing Office Washington, DC).
National Geophysical Data Center / World Data Service (NGDC/WDS). 2017.
Global Historical Tsunami Database. National Geophysical Data Center, NOAA. doi:10.7289/V5PN93H7
Russel, I. C. 1893.
Malaspina Glacier. The Journal of Geology 1: 3.
Wiles, G. C. and P. E. Calkin. 1992.
Reconstruction of a debris-slide-initiated flood in the southern Kenai Mountains Alaska. Geomorphology 5: 535–546.
Last updated: December 30, 2019