Many [ecosystem] services … are directly generated by protected park ecosystems … and provide significant benefits beyond park borders. These … contributions are little studied … but can be crucial sources of services required for human well-being.
Get PDF
Download PDF of this article
Abstract and key words
Abstract: Protected areas such as national parks are recognized as important providers of ecosystem services, the benefits nature conveys to humans. However, some threats to these services, such as air pollution, can derive from outside a park’s boundaries. Ground-level ozone (O3) is a human-made pollutant that at elevated levels can damage vegetation, resulting in decreased growth and increased water loss through evapotranspiration, which in turn results in decreased overall streamflow. Using studies conducted on similar ecosystems in and near Great Smoky Mountains National Park, we estimated the potential loss from O3 damage of two ecosystem services, climate regulation (through the intermediate service of carbon sequestration) and water provisioning (through streamflow), at this national park. These ecosystem functions directly benefit humans by providing a livable climate and by providing downstream beneficiaries with water for drinking, agriculture, recreation, and hydropower. We found the loss from impairment of these services could be significant when O3 levels are elevated. A 50% increase in O3 exposure is projected to result in a loss of carbon (C) sequestration of 500,000–960,000 t C yr−1 (metric tons of C per year) (551,000 sh t C yr−1), while a 25% reduction in O3 concentrations could result in an increase in streamflow of 109.6 M m³ (million cubic meters) (88,854 ac-ft) from the park during the critical dry August–October period. This highlights the important services provided by protected landscapes such as this national park and the need for more in-depth research on the effects air pollution can have on the benefits we receive from nature.
Key Words: air pollution, carbon sequestration, ecosystem services, Great Smoky Mountains, ozone, water provision
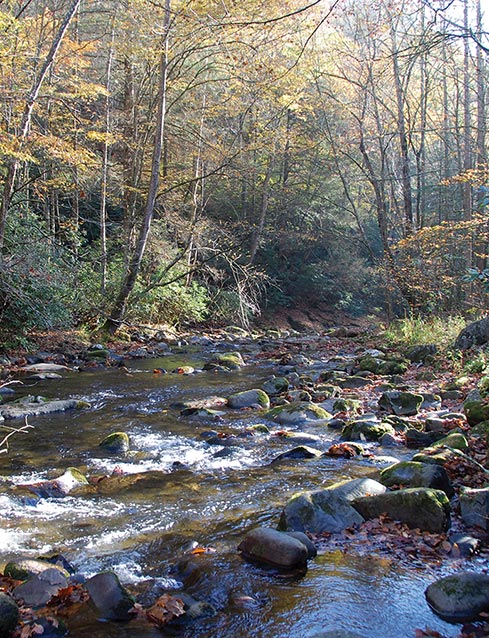
NPS/Tamara Blett
THE ASSESSMENT OF ECOSYSTEM SERVICES IS IMPORTANT for characterizing the benefits nature provides to humans, and facilitates the use of ecosystem indicators in public decision making (Patterson and Coelho 2009). Ecosystem services have been defined as “components of nature, directly enjoyed, consumed or used to yield human well-being” (USEPA 2013a, 3). Some ecosystem services, such as crop pollination and flood protection, are well documented and are fairly straightforward to quantify; others, such as scenic vistas, are less tangible yet no less important to human well-being. As protected areas, the National Park System is ideally situated to provide many services that are beneficial to humans. Traditionally parks have been valued for their aesthetic, spiritual, and cultural contributions to human welfare. Using the ecosystem services framework, however, we now realize that while many services, such as climate regulation or water provision, are directly generated by protected park ecosystems, they flow outside park boundaries and provide significant benefits beyond park borders (fig. 1). These external contributions are little studied, if at all, but can be crucial sources of services required for human well-being.
Although national parks are among the most protected landscapes in the country, some threats do not respect boundaries. Air pollution may principally originate outside a park but can damage park ecosystems and the services these ecosystems provide if levels are too high. One such pollutant is tropospheric ozone (O3), which has long been known to damage vegetation and is still considered to be one of the most harmful air pollutants to forests and rural areas (figs. 2 and 3, below) (Ashmore 2005; Paoletti et al. 2010). It is formed via a complex set of atmospheric reactions in the presence of sunlight as a secondary reaction; generally its precursors are oxidized nitrogen (NOx) and volatile organic compound (VOC) emissions from stationary and mobile sources (Finlayson-Pitts and Pitts 1993). Over the past 20 years there has been a shift in research on the effects of air pollution on forests from a focus on forest health and effects on forest production to a focus on ecosystem services (Paoletti et al. 2010). Much of the focus of air pollution effects on ecosystem services provided by forests has been on changes to intermediate services such as carbon (C) sequestration or nitrogen effects, which can affect climate regulation and biodiversity; however, forest ecosystems are also important sources of other services, including provision of water for human consumption, agricultural and recreational uses, and hydropower production (Compton et al. 2011).
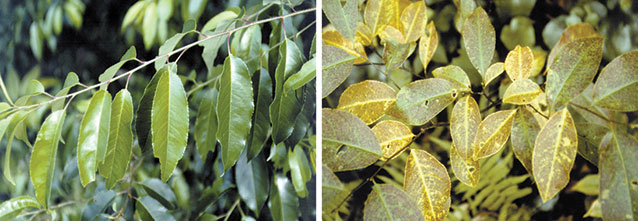
NPS/Jim Renfro
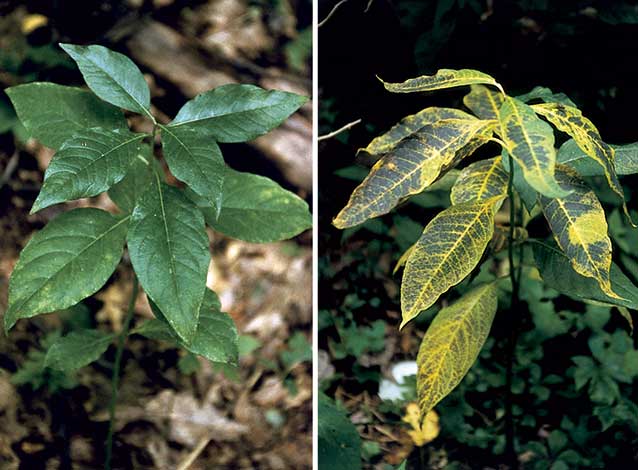
NPS/Jim Renfro
Great Smoky Mountains National Park (hereafter “the park”), incorporating approximately 210,500 ha (520,157 ac), is located in Tennessee and North Carolina. The headwaters for 45 watersheds are located in the park and half of the old-growth forest still present in the eastern United States is located within its boundaries. It has more species of native vascular plants than any other park in North America and more tree species than all of northern Europe (NPS 2002). High levels of tropospheric O3 have been a concern at the park since the 1970s and can be double those in the nearby cities of Knoxville and Atlanta (NPS 2011). In 2011, O3 at the park remained at elevated levels associated with demonstrated damage to vegetation (NPS 2013).
We examined two ecosystem services: C sequestration and drinking water provision. Carbon sequestration in biomass has been identified as a key strategy for mitigating the effects of climate change caused by anthropogenic additions of carbon dioxide (IPCC 2014). Provision of water for extractive purposes such as municipal, agricultural, and commercial use, and in situ uses such as hydropower production, recreation, and transportation, is a vital benefit provided by ecosystems (Brauman et al. 2007). We used data quantifying changes to tree growth and stream base flow because of O3 damage to vegetation obtained at a nearby study area to estimate effects on these ecosystem services provided by the park as a whole.
The ecosystem services framework provides an easily understood way for policymakers and nonscientists to conceptualize the important benefits nature provides and to assess the effects external influences can have on protected areas. By translating empirical data concerning effects of air pollution on vegetation to effects on ecosystem services, land managers and policymakers will be able to better incorporate the benefits provided by protected areas in their decisions. Though this approach may not be as robust as direct measurement or modeling, these calculations set the stage and demonstrate the need for further research to better assess the effects of air pollution on ecosystem services.
Carbon sequestration
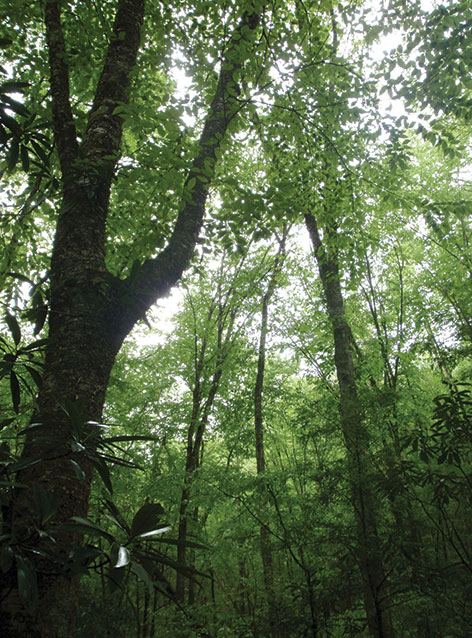
NPS/Colleen Flanagan Pritz
We used existing data from studies of changes to tree growth because of O3 exposure to estimate reduction in carbon sequestration. Ozone has the effect of reducing carbon storage by reducing the net primary production (NPP) of vegetation (Felzer et al. 2002) (fig. 4). McLaughlin et al. (2007a) found that high levels of O3 can limit the growth of mature forest trees because of chronic alterations in photosynthetic production and carbon allocation, and increased levels of water stress through increased transpiration. Stem increment and sap flow velocity were measured in a variety of tree species over three sites in eastern Tennessee, including the park. McLaughlin et al. (2007a) compared responses between two years with average cumulative seasonal O3 exposure >60 parts per billion (ppb) and one year with elevated O3 cumulative exposure (50% higher). They found that elevated levels of ambient O3 exposure were associated with growth reductions of 30–50% (averaging about 40%). Similar reductions in angiosperm biomass were reported by Wittig et al. (2009) for O3 concentrations above 80 ppb.
Because the McLaughlin et al. study used tree stem increment measurements to obtain changes in rates of growth because of O3, we used measurements of aboveground net primary production (ANPP) to obtain carbon-budget estimates for southeastern U.S. deciduous forests. Busing (2005) used tree growth increments and relationships between characteristic tree dimensions in the Great Smoky Mountains, Tennessee, to estimate ANPP and found that a range of 630–860 g C m−2 yr−1 (grams of carbon per square meter per year) (18–25 oz C yd−2 yr−1) is accumulated in old stands of temperate deciduous forest similar to those found in the park (table 1). We also used results from two modeling studies to inform our range of ANPP. Curtis et al. (2002) used biometric as well as eddy covariance methods to estimate NPP for several sites. The eastern Tennessee deciduous forest site in this study had an estimated ANPP of 446 g C m−2 yr−1(13 oz C yd−2 yr−1) (wood + leaves portion of their NPP estimate). Zhang et al. (2007) used the Dynamic Land Ecosystem Model to estimate carbon storage in the park. Their results revealed an NPP contribution of 748 g C m−2 yr−1 (22 oz C yd−2 yr−1). They assumed a belowground-to-aboveground NPP ratio of 0.4, which resulted in an estimated ANPP of 449 g C m−2 yr−1 (13 oz C yd−2 yr−1).
Table 1. Range of carbon sequestration losses with 40% reduction in aboveground net primary production | |||||
---|---|---|---|---|---|
Study | ANPPg C m−2 yr−1 | GRSM t yr−1 | Is 40% of (t) | Difference (t) | Equal to Cars yr−1 |
Busing (2005) | 860 | 1,437,760 | 2,396,266 | 958,506 | 688,686 |
Curtis et al. (2002) | 446 | 745,629 | 1,242,714 | 497,085 | 357,156 |
Notes: |
Based on these studies of C accumulation in eastern Tennessee deciduous forests, we examined a range of ANPP values, using the value of 446 g C m-2 yr-1 (13 oz C yd-2 yr-1) as a lower bound and 860 g C m-2 yr-1(25 oz C yd-2 yr-1) as an upper bound for estimates of C sequestration in the park. Deciduous forest in the park comprises an area of 167,181 ha (413,114 ac). Under the assumption that all deciduous forest adds biomass at the same rate, we estimate from the ANPP values that 0.7–1.4 M t C yr-1 (million metric tons of carbon per year) (0.8–1.5 M short tons [sh t] C yr-1) is added as biomass to the aboveground vegetation in the park. Under conditions of elevated cumulative O3 exposure, these ANPP values would represent a 40% reduction in growth according to McLaughlin et al.’s observations. Therefore, carbon sequestration without O3 damage could be about 1.2–2.4 M t C yr-1 (1.3–2.6 M sh t C yr-1). The difference from the current level of C sequestration, and consequently the lost C sequestration in the park deciduous forest in a year with 50% above average O3 exposure, could be in the range of 500,000–960,000 t C yr-1 (551,000–1,057,920 sh t C yr-1) because of the growth effects of O3 damage.
Carbon sequestration discussion
Damage to vegetation from enhanced levels of O3 has been documented in Great Smoky Mountains National Park (Neufeld et al. 1991; Chappelka et al. 1999, 2007) and results in lowered amounts of biomass accumulation (McLaughlin et al. 2007b) (see figs. 2 and 3). Our climate has unequivocally been shown to be warming because of observed increases in anthropogenic greenhouse gases, especially carbon dioxide (CO2) from fossil fuel burning (IPCC 2013). Trees can act as a sink for CO2 by fixing carbon during photosynthesis and storing excess carbon as biomass (Nowak and Crane 2002) and can store large amounts of carbon for long time periods (Heath et al. 2003). About 20% of the world’s plant biomass can be found in temperate forests such as those found in the park, and mature temperate forests have high rates of C sequestration (Bonan 2008). Ozone can act to reduce carbon storage by reducing net primary production, and on a worldwide scale has been found to account for a reduction of carbon storage of about 800–1,300 M t C yr−1 (882–1,433 M sh t C yr−1) (Felzer et al. 2005).
The southeastern United States has been identified as an especially important region for C sequestration because it is an area where high O3 levels coincide with high plant productivity (Felzer et al. 2005). Applying the growth reduction estimate of ~40% in deciduous forest found by McLaughlin et al. (2007b) to the ANPP estimates of biomass accumulation, we find that approximately 500,000–960,000 t C yr−1(551,000–1,057,920 sh t C yr−1) of C sequestration can be lost during a year with high cumulative O3exposure. To put this in context, according to the U.S. Environmental Protection Agency, the average vehicle in the United States emitted 5.1 t (metric tons) (5.6 sh t) of CO2 in 2007 (USEPA 2012). Using this figure, the amount of lost C sequestration is similar to the amount of carbon emitted by about 360,000 to 690,000 vehicles per year.
Our analytical approach documents an effective method for translating growth reductions because of O3damage to effects on ecosystem services, and finds that in an anomalously high year of O3 exposure, when cumulative O3 exposure exceeds the average by 50% and hourly maximum values reach 120 ppb, significant losses of C sequestration can occur. While this is a relatively uncommon level, the park has experienced elevated levels of O3 as recently as 2012 (National Park Service, B. Sive, atmospheric chemist, personal communication, Lakewood, Colorado, 29 May 2014), and the large amount of lost C sequestration at this exposure suggests that even at lower O3 levels there is likely to be a substantial loss of C sequestration capacity. A meta-analysis by Wittig et al. (2009) found growth reductions of 11–17% at an elevated O3exposure average of 97 ppb. Even at average background O3 levels of 40 ppb across the 263 studies, researchers found a reduction in tree biomass of 7%. These widely documented reductions in photosynthetic capacity and plant growth suggest that even with a large amount of uncertainty there is likely to be a substantial negative effect on C sequestration at more typical O3 levels (USEPA 2013b).
Water provisioning
We also examined how previous study results could be employed to obtain estimates of changes to water provision because of O3 damage to vegetation. High levels of O3 exposure, especially acute exposure at high concentrations, have been found to have several harmful effects on vegetation, including the onset of sluggish stomatal responses to stimuli (McLaughlin et al. 2007b) (see figs. 2 and 3). This can lead to incomplete closure of the stomata, weakening control of water loss that can persist long after O3 exposure, and may lead to increased evaporation of water from leaves as the stomata fail to close (McLaughlin et al. 2007b; Paoletti 2007). This increased water use and loss through the leaves causes reduced soil moisture and reductions in streamflow in the watershed as trees increase their uptake of water to offset the loss. Using a stepwise regression model, McLaughlin et al. (2007b) predicted that a 25% reduction in O3 would increase watershed base flow by 62% during the dry August–October period and would result in a long-term annual average increase in streamflow of around 5%, as less soil water would be lost through vegetation.
We evaluated the potential consequences of an O3-induced decrease in streamflow on an area of the park. The U.S. Geological Survey (USGS) stream gauge 03497300 is located near the park boundary and records outflow from a drainage area of approximately 27,500 ha (67,954 ac) (USGS 2012), nearly all of which is located within two watersheds in the park (fig. 5). Mean annual volume of water passing this gauge for the period 1964–2010 is 251 million m³, which is more than 66 billion gallons, or 203,488 ac-ft. During the August–October period the mean total flow passing the gauge is 30,866,988 m³ (25,024 ac-ft) (table 2). McLaughlin et al. (2007a) estimated that during this period 95% of the streamflow (29,323,638 m³ or 23,773 ac-ft) comprises base flow. Using ESRI’s ArcGIS 10.1 software and the USGS 2006 National Land Cover Database (NLCD), we have calculated that deciduous forest occupies an area of approximately 24,000 ha (59,305 ac) of the 27,500 ha (ArcGIS NLCD value; 67,954 ac) of the two watersheds that drain past this stream gauge, or about 88% of the land area.
Table 2. Streamflow at USGS gauge 03497300, Little River, above Townsend, Tennessee | |||
---|---|---|---|
Month | Mean CFS | Seconds mo−1 | M3 mo−1 |
August | 162 | 2,678,400 | 12,288,071 |
September | 126 | 2,592,000 | 9,249,085 |
October | 123 | 2,678,400 | 9,329,831 |
August–October | N/A | N/A | 30,866,987 |
Note: CFS = cubic feet per second. |
We assumed an even distribution of precipitation across these two watersheds during the 1964–2010 period from which the stream gauge average is computed. Since the McLaughlin et al. (2007a) study examined base flow effects in deciduous forests, we calculated that approximately 25.7 M m³ (20.8 M ac-ft) (88%) of base flow would originate in deciduous forest areas of the two watersheds during the August–October period when O3 exposure is at average levels. McLaughlin et al. (2007a) predicted that if O3 were decreased 25% from average-year concentrations, an increase in streamflow of 62% could occur; this would increase flow to 41.5 M m³ (33.6 M ac-ft) at the stream gauge. The difference, meaning the missed base flow as a result of O3 damage to vegetation, is about 15.8 M m³ (12.8 M ac-ft) over these two watersheds (table 3). Over the entire park area (1,671,813,900 m² 167,814 ha [413,114 ac] of deciduous forest according to NLCD) this would indicate a lost base flow of 109.6 M m³ (88,854 ac-ft) over the August–October period.
Table 3. Base flow reduction due to ozone-damaged vegetation | |||
---|---|---|---|
Parameter | Volume (m3) | Comparison | Metric |
August–October streamflow | 30,866,987 | ||
August–October base flow | 29,323,638 | ||
Base flow originating in deciduous in-stream gauged watersheds | 25,707,169 | ||
Deciduous base flow (m3) per m2 in gauged watersheds | 0.11 | Alcoa resident average water usage | 73.3 m³ mo−1 |
62% of base flow originating in deciduous watersheds | 41,463,175 | ||
Difference between measured deciduous base flow and 100% | 15,756,006 | Equivalent Alcoa residential customers | 71,629 |
Deciduous base flow in GRSM | 178,751,369 | ||
62% of deciduous base flow in GRSM | 288,318,357 | ||
Difference between measured deciduous GRSM base flow and 100% | 109,566,988 | Equivalent Alcoa residential customers | 498,106 |
Water provisioning discussion
Provisioning of water is one of the most important services ecosystems provide to humans (Brauman et al. 2007), and high O3 exposure may indirectly threaten water delivery from Great Smoky Mountains National Park. Forest ecosystems are the most important source of water in the United States, providing 53% of the nation’s water supply and about 62% of the water supply in North Carolina and Tennessee (Brown et al. 2008). Climate change may exacerbate stress on water resources because of increased precipitation variability, and it may change seasonal timing of runoff (IPCC 2013). This could act to intensify changes in streamflow from O3, as the cumulative seasonal effects of O3 exposure on base flow are expected to have the greatest impact during the late-season low-flow period (McLaughlin et al. 2007b). Base flow is one aspect of the flow regime that is critical for regulating biotic production and diversity, and reductions are expected to have effects on in-stream nutrient concentrations, water temperatures, and dissolved oxygen levels (Baron et al. 2002; McLaughlin et al. 2007b; Baron et al. 2013).
In addition to ecological consequences, streamflow originating in the park is appropriated for human uses downstream. Many rivers and streams originating in the park drain into impoundments where the water is used for agriculture, recreation, and hydropower. Much of eastern Tennessee relies on both groundwater and surface water as sources of drinking water. Maryville, for example, a city of 27,000, relies on the Little River (approximately 30 km [19 mi] downstream from the USGS gauge used in this analysis) for its drinking water. Some of these cities, including Maryville, have identified drought as a potential threat, even implementing mandatory water restrictions in the past (Tennessee Department of Environment and Conservation 2009).
By applying McLaughlin et al.’s (2007a) findings of a 62% reduction of stream base flow during the crucial dry August–October period, we find that 109.6 M m³ (88,854 ac-ft) of lost base flow is possible over the entire park because of damage to vegetation when O³ levels are elevated by 25%. Alcoa, Tennessee, is downstream from the Little River USGS gauge and draws its water from the Little River (City of Alcoa 2007). An average resident uses approximately 73.3 m³ mo−1 (19,364 gal/month) of water (City of Alcoa 2007). The amount of stream base flow reduction because of O3 damage to vegetation in the park is approximately equal to the amount of water used by 498,000 residents of Alcoa during an average three-month period. A subsequent study found similar reductions in streamflow in high O3 years over a wide geographic area of the southern Appalachian Mountains consisting of many tree species (Sun et al. 2012). This indicates that O3 could be an important influence on streamflow and ecosystem services over a large area, and could act to exacerbate drought conditions.
Conclusions
As permanently protected areas, national parks are important providers of ecosystem services that benefit humans in multiple ways. However, threats to these services often come from outside the park’s borders and are beyond the control of the National Park Service. The benefits from nature preserved in national parks can be great, yet decision makers often lack information on how people benefit from specific ecosystem services (Turner and Daily 2008; Compton et al. 2011). Resource preservation and protection are often overlooked policy objectives, yet these objectives can be justified powerfully by consideration of ecosystem services and the benefits they provide (Salzman et al. 2001). This is especially true for O3, as the long-range transport of O3 can result in elevated levels in rural protected areas far from emissions sources (Adams et al. 1986).
Although this analysis entails a greater level of uncertainty in results because of the limited number of studies used to estimate the effects on changes to ANPP and especially stream base flow (and assumptions about the transfer of effects to the ecosystem scale), it nonetheless demonstrates a fast, straightforward, and easily comprehensible method for determining effects on ecosystem services. We found that injury to vegetation when O3 levels are 50% above normal is projected to decrease carbon sequestration across the park by 500,000–960,000 t C yr−1 (551,000–1,057,920 sh t C yr−1), the amount of carbon added to the atmosphere by 360,000–690,000 cars in a year. In addition we found that reducing O3 levels by 25% could increase streamflow originating in the park by 109.6 M m3 (88,854 ac-ft) during the dry August–October period. This is the equivalent water use of approximately 500,000 municipal consumers at a time when water demand by both humans and ecosystems is often at its peak but supplies are often reduced.
The potential magnitude of losses draws attention to the urgent need for further investigation and more rigorous estimates of loss of ecosystem services and human well-being that is incurred from continued O3pollution. Connecting the environmental consequences of air pollution (effects on tree growth, water loss, and streamflow) to the human beneficiaries of these services is a crucial link, especially since intermediate ecosystem services such as C sequestration are often underappreciated components of final ecosystem services (USEPA 2013a). Framing this link in a manner that is accessible to policymakers, such as putting it in terms of automobile emissions or municipal water customers, is an important step toward communicating the damage suffered by ecosystem services from O3 incursion and the benefits of implementing mitigation measures.
Managing ecosystem services will become increasingly important as the human footprint on the landscape continues to grow (Kreman 2005). Raising the awareness of policymakers about how their decisions affect how humans can benefit from nature, even when these benefits originate in otherwise protected areas such as national parks, is crucial to their preservation and the human well-being associated with those services. Quantifying the deleterious effects of O3 pollution on ecosystem services and putting them in a framework that can easily be understood and used to guide policy decisions are an important component of pollution mitigation strategies.
References
Adams, R. M., S. A. Hamilton, and B. A. McCarl. 1986. The benefits of pollution control: The case of ozone and U.S. agriculture. American Journal of Agricultural Economics 68:886–893.
Ashmore, M. R. 2005. Assessing the future global impacts of ozone on vegetation. Plant, Cell, and Environment 28:949–964.
Baron, J. S., E. K. Hall, B. T. Nolan, J. C. Finlay, E. S. Bernhardt, J. A. Harrison, F. Chan, and W. Boyer. 2013. The interactive effects of excess reactive nitrogen and climate change on aquatic ecosystems and water resources of the United States. Biogeochemistry 114:71–92. doi:10.1007/s10533-012-9788-y.
Baron, J. S., N. L. Poff, P. L. Angermeier, C. N. Dahm, P. H. Gleick, N. G. Hairston, R. B. Jackson, C. A. Johnston, B. D. Richter, and A. D. Steinman. 2002. Meeting ecological and societal needs for freshwater. Ecological Applications 12:1247–1260.
Bonan, G. B. 2008. Forests and climate change: Forcings, feedbacks, and the climate benefits of forests. Science 320:1444–1449.
Brauman, K. A., G. C. Daily, T. K. Duarte, and H. A. Mooney. 2007. The nature and value of ecosystem services: An overview highlighting hydrologic services. Annual Review of Environment and Resources 32:67–98.
Brown, T. C., M. T. Hobbins, and J. A. Ramirez. 2008. Spatial distribution of water supply in the conterminous United States. Journal of the American Water Resources Association 44:1474–1487.
Busing, R. T. 2005. NPP temperate forest: Great Smoky Mountains, Tennessee, U.S.A., 1978–1992. Data set. Oak Ridge National Laboratory Distributed Active Archive Center, Oak Ridge, Tennessee, USA. Available at http://www.daac.ornl.gov.
Chappelka, A., J. Skelly, G. Somers, J. Renfro, and E. Hildebrand. 1999. Mature black cherry used as a bioindicator of ozone injury. Water, Air, and Soil Pollution 116:261–266.
Chappelka, A. H., G. L. Somers, and J. R. Renfro. 2007. Temporal patterns of foliar ozone symptoms on tall milkweed (Asclepias exalta L.) in Great Smoky Mountains National Park. Environmental Pollution 149:358–365.
City of Alcoa. 2007. Public works statistical snapshot. Accessed 15 October 2012 at http://www.cityofalcoa-tn.gov/content/view/full/699.
Compton, J. E., J. A. Harrison, R. L. Dennis, T. L. Greaver, B. H. Hill, S. J. Jordan, H. Walker, and H. V. Campbell. 2011. Ecosystem services altered by human changes in the nitrogen cycle: A new perspective for U.S. decision making. Ecology Letters 14:804–815.
Curtis, P. S., P. J. Hanson, P. Bolstad, C. Barford, J. C. Randolph, H. P. Schmid, and K. B. Wilson. 2002. Biometric and eddy-covariance based estimates of annual carbon storage in five North American deciduous forests. Agricultural and Forest Meteorology 113:3–19.
Felzer, B., D. W. Kicklighter, J. M. Melillo, C. Wang, Q. Zhuang, and R. Prinn. 2002. Ozone effects on net primary production and carbon sequestration in the conterminous United States using a biogeochemistry model. Report No. 90. MIT Joint Program on the Science and Policy of Global Change. Cambridge, Massachusetts, USA.
Felzer, B., J. Reilly, J. Melillo, D. Kicklighter, M. Sarofim, C. Wang, R. Prinn, Q. Zhuang. 2005. Future effects of ozone on carbon sequestration and climate change policy using a global biogeochemical model. Climate Change 73:345–373.
Finlayson-Pitts, B. J., and J. N. Pitts Jr. 1993. Atmospheric chemistry of tropospheric ozone formation: Science and regulatory implications. Journal of the Air and Waste Management Association 43:1091–1100.
Heath, L. S., J. E. Smith, and R. A. Birdsey. 2003. Carbon trends in U.S. forestlands: A context for the role of soils in forest carbon sequestration. Pages 35–45 in J. M. Kimble, L. S. Heath, R. A. Birdsey, and R. Lal, editors. The potential of U.S. forest soils to sequester carbon and mitigate the greenhouse effect. CRC Press, Boca Raton, Florida, USA.
International Panel on Global Climate Change (IPCC). 2013. Climate change 2013: The physical science basis. Working Group I report. IPCC Secretariat, Geneva, Switzerland.
———. 2014. Climate change 2014: Mitigation of climate change. Working Group III report. IPCC Secretariat, Geneva, Switzerland.
Kremen, C. 2005. Managing ecosystem services: What do we need to know about their ecology? Ecology Letters 8:468–479.
McLaughlin, S. B., M. Nosal, S. D. Wullschleger, and G. Sun. 2007a. Interactive effects of ozone and climate on tree growth and water use in a southern Appalachian forest in the USA. New Phytologist 174:109–124.
McLaughlin, S. B., S. D. Wullschleger, G. Sun, and M. Nosal. 2007b. Interactive effects of ozone and climate on water use, soil moisture content and streamflow in a southern Appalachian forest in the USA. New Phytologist 174:125–136.
National Park Service (NPS). 2002. Air quality in the national parks. Second edition. National Park Service, Air Resources Division, Lakewood, Colorado, USA.
———. Air pollution impacts, Great Smoky Mountains National Park. Last modified 17 August 2011. Accessed 15 October 2012 at https://www.nature.nps.gov/air/Permits/ARIS/grsm/impacts.cfm?tab=1#TabbedPanels1.
———. 2013. Annual data summary 2011, Gaseous Pollutant Monitoring Program. Natural Resource Data Series NPS/NRSS/ARD/NRDS-2011/192. National Park Service, Natural Resource Stewardship and Science, Denver, Colorado, USA.
Neufeld, H. S., J. R. Renfro, W. D. Hacker, and D. Silsbee. 1991. Ozone in Great Smoky Mountains National Park: Dynamics and effects on plants. Pages 594–617 in R. L. Berglund, editor. Tropospheric ozone and the environment II. Air and Waste Water Management Association, Pittsburgh, Pennsylvania, USA.
Nowak, D. J., and D. E. Crane. 2002. Carbon storage and sequestration by urban trees in the USA. Environmental Pollution 116:381–389.
Paoletti, E. 2007. Ozone impacts on forests. CAB Reviews: Perspectives in Agriculture, Veterinary Science, Nutrition and Natural Resources 068:2–13.
Paoletti, E., M. Schaub, R. Matyssek, G. Wieser, A. Augustaitis, A. M. Bastrup-Birk, A. Bytnerowicz, M. S. Günthardt-Goerg, G. Müller-Starck, and Y. Serengil. 2010. Advances of air pollution science: From forest decline to multiple-stress effects on forest ecosystem services. Environmental Pollution 158:1986–1989.
Patterson, T. M., and D. L. Coelho. 2009. Ecosystem services: Foundations, opportunities, and challenges for the forest products sector. Forest Ecology and Management 257:1637–1646.
Salzman J., B. H. Thompson Jr., and G. C. Daily. 2001. Protecting ecosystem services: Science, economics, and law. Stanford Environmental Law Journal 20:309–332.
Sun, G. E., S. B. McLaughlin, J. H. Porter, J. Uddling, P. J. Mulholland, M. B. Adams, and N. Pederson. 2012. Interactive influences of ozone and climate on streamflow of forested watersheds. Global Change Biology 18:3395–3409.
Tennessee Department of Environment and Conservation. 2009. Protection of potable water supplies in Tennessee watersheds. Tennessee Department of Environment and Conservation, Division of Water Pollution Control and Division of Water Supply, Nashville, Tennessee, USA.
Turner, R. K., and G. C. Daily. 2008. The ecosystem services framework and natural capital conservation. Environmental and Resource Economics 39:25–35.
U.S. Environmental Protection Agency (USEPA). 2012. Calculations and references, passenger vehicles per year. Last modified 31 May 2012. Accessed 15 October 2012 at http://www.epa.gov/cleanenergy/energy-resources/refs.html.
———. 2013a. Final ecosystem goods and services classification system (FEGS-CS). EPA/600/R-13/ORD-004914. Corvallis, Oregon, USA.
———. 2013b. Integrated science assessment for ozone and related photochemical oxidants. EPA/600/R-10/076F. Research Triangle Park, North Carolina, USA.
U.S. Geological Survey (USGS). 2012. Water data report 2010, 03497300, Little River above Townsend, TN. Accessed October 2012 at https://wdr.water.usgs.gov/wy2010/pdfs/03497300.2010.pdf.
Wittig, V. E., E. A. Ainsworth, S. L. Naidu, D. F. Karnosky, and S. P. Long. 2009. Quantifying the impact of current and future tropospheric ozone on tree biomass, growth, physiology and biochemistry: A quantitative meta-analysis. Global Change Biology 15:396–424.
Zhang, C., H. Tian, A. H. Chappelka, W. Ren, H. Chen, S. Pan, M. Liu, D. M. Styers, G. Chen, and Y. Wang. 2007. Impacts of climatic and atmospheric changes on carbon dynamics in the Great Smoky Mountains National Park. Environmental Pollution 149:336–347.
About the Authors
Andrew Bingham is an ecologist with the NPS Air Resources Division. Ellen Porter, biologist, recently retired from the NPS Air Resources Division.
Documentation
Suggested citation for this article
Bingham, A., and E. Porter. 2015. Ozone effects on two ecosystem services at Great Smoky Mountains National Park, USA. Park Science 32(1):71–79.
This article published
Online: 4 September 2015; In print: 14 September 2015
URL
https://www.nps.gov/articles/parkscience_32_1_71-79_bingham_porter_3825.htm
This page updated
16 September 2015
Site navigation
Last updated: August 9, 2018