Part of a series of articles titled Yellowstone Science - Volume 27 Issue 1: Vital Signs - Monitoring Yellowstone's Ecosystem Health.
Article
Nowcasting & Forecasting Fire Severity in Yellowstone
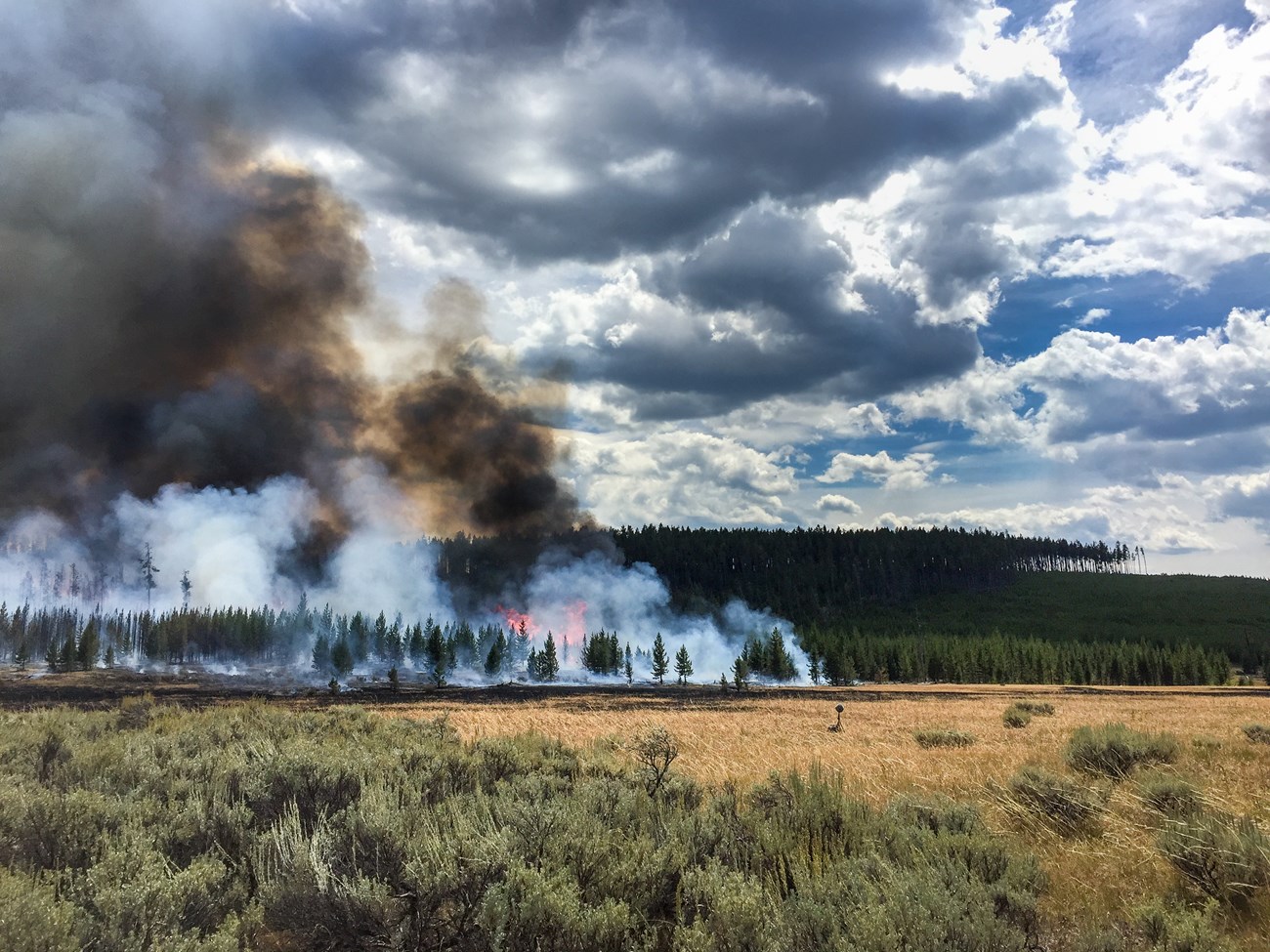
Nowcasting & Forecasting Fire Severity in Yellowstone
by Mike Tercek
Fire Severity is Increasing
Climb any mountain in the spring, and you will find that Yellowstone National Park (YNP) is made almost entirely in shades of green. Many grayish-blue peaks encircle the far horizon; but in the park itself, only a few nunatak mountains push up pinpoints of bare rock. Thick, green forests cover 80% of the landscape. Grassy valleys and sagebrush fill in most of the rest (Despain 1990). Yellowstone is defined by its plants. Most of Yellowstone’s scenery—and habitat—is the result of a living, green adaptation to fire.
Many plants in YNP are adapted to fire, but probably none more so than the lodgepole pine that dominates the central plateaus. During the last 2,000 years, lodgepole forests in YNP have experienced large (>6,175 acres; >2,500 ha;), stand-replacing fires every 200-400 years (Romme and Despain 1989, Millspaugh et al. 2000). This regular fire cycle, combined with the nutrient-poor volcanic soil inside the caldera, has given the lodgepole an advantage over species that would otherwise be better adapted to the climate (Despain 1990). If fire were to become less frequent, many lodgepole stands would be replaced by spruce and fir. If fire became more common, grassland and sagebrush steppe would gain the advantage.
In recent years, human-caused climate change has increased fire frequency and severity across the western United States, and computer-driven climate models forecast even more rapid and severe increases in the future (Westerling et al. 2006, Westerling et al. 2011, Abatzoglou and Williams 2016, Westerling 2016, Clark et al. 2017). During the period 2003-2012, the northern Rocky Mountains had 889% more fires and 2,699% more acres burned than during the period 1973-1982. Most of these increases were linked to snow melting earlier in the year, which increases drought and the length of the fire season (Westerling 2016). Climate models forecast that by the mid-21st century, the annual area burned in YNP will exceed 247,000 acres (100,000 ha); this represents approximately 11% of the total area of the park burned during most years. The climate will be as dry as 1988, the biggest fire year in recent history, every five years or less. If the models are accurate, the time required to burn an area equivalent in size to the entire park will decrease to less than 30 years (Westerling et al. 2006, Westerling et al. 2011).
The recently observed fire increases and the future forecasts are both driven by the strong relationship between drought and fire, but there is some debate over whether fire increases will continue to keep pace with drought increases in the future. Critics point out that drought is only one of the ingredients needed for fire. Fire also needs fuel (forests) to burn; and once a large portion of the forests have been burned, less fuel will be available. The link between drought and fire may weaken, potentially making future fire increases less severe than forecasted by the models (McKenzie and Littell 2017).
Even if the climate model forecasts are overestimating fire potential—making the droughty future seem perhaps twice as fiery as it will in fact be—there is little doubt that YNP’s defining greenness will change dramatically in the coming century. There will either be fewer forests or different kinds of forests. If climate continues to be the factor controlling fire, then forests will burn so frequently that lodgepole pine will not have time to reproduce and will be replaced by grasslands, sagebrush, or more fire-resistant Douglas-fir on the central plateaus (Westerling et al. 2011, Clark et al. 2017). If instead fuel becomes the limiting factor, fire activity will decrease only after most of the forests (i.e., fuel) have been burned off. Future fire is also likely to interact with other disturbances that further reduce the size and density of the park’s forests. Tree cover will likely decrease in YNP as the “climate envelope”—the combinations of temperature and precipitation that forests require—becomes less common on the landscape (Hansen et al. 2015). At the same time, warming temperatures are likely to increase the frequency and severity of tree-killing beetle outbreaks (Logan et al. 2010, Mitton and Ferrenberg 2012).
As fire danger reaches unprecedentedly high levels in the future, managers will need tools that help them quickly analyze large amounts of data and make high-stakes decisions regarding ecosystem health and human safety. Since fire danger often changes rapidly in a single day and can vary greatly from place to place (figure 1), our responses need to be adaptable and quickly evolving. In this article, I describe a recently-developed, web-based tool that automatically collects data from 15 different weather stations in YNP and presents fire danger in an easy to interpret format. This tool produces results similar to those developed by the authors described above (e.g., Westerling 2006, Westerling et al. 2011); however, it has the advantage of being much simpler and less resource-intensive to compute, making it practical as a source of “nowcasts” or present predictions based on data that can be updated near real-time. I also present estimates of mid- to late 21st century fire severity in YNP, which were obtained by applying the web-based tool to future climate data from downscaled general circulation models.
Nowcasting Today’s Fire Danger
The model I developed to nowcast daily fire danger used an “elastic net” linear regression (Zou and Hastle 2005, Bowles 2015). This regression used 83 climate measurements from 15 weather stations to predict the number of acres that would be burned if a fire were left unchallenged. As part of model development, I started with an initial set of 1,150 candidate climate measurements that were then reduced to the 83 “best” predictors. A publication on the development of this model is currently in preparation.All the climate measurements were taken from snow telemetry stations (SNOTEL; www.wcc.nrcs.usda.gov/snow/) because these stations have few missing values and better quality hydrology parameters than other weather station types. In addition to standard measurements of snow, temperature, and precipitation, I also included calculated parameters such as the Keetch-Byram Drought Index (Keetch and Byram 1968, Alexander 1990) and parameters estimated from a water balance model (Thornthwaite and Mather 1955, Lutz et al. 2010) such as moisture deficit and evapotranspiration.
Once the final regression model was created, it was coded as a module for www.ClimateAnalyzer.org, which delivers climate data summaries and products based on automatically updated, real-time data. An easy way to interpret the model would be as follows: based on 83 predictor variables, I calculate conditions today are as dry as the driest conditions during year X, when Y acres burned. For example, if the elastic net regression determines conditions are nearly as dry as those seen during the height of the fire season in 1988, then the regression will report an estimated fire danger of roughly 800,000 acres (323,749 ha) or 36% of the entire park area. The scale is calculated by the regression on a continuous scale, so any level of fire danger is possible.
Forecasting Future Fire Danger
In order to forecast fire danger for the mid- and late 21st century, the elastic net regression was applied to future climate data derived from three computer-driven, global climate models (Rupp et al. 2013, Thrasher et al. 2013). The times series extracted from the future models was specific to the same 15 SNOTEL station locations that were used for nowcasting, so that the elastic net regression could be used without changes. For more detail on data sources and methodology, including the estimation of snow parameters in the future data, see Tercek and Rodman (2016).Fire danger was initially estimated on a daily time-step for the years 2031-2099 and then summarized as 10-year sums (i.e., acres burned per decade). The analysis focused on two climate scenarios or representative concentration pathways (RCPs; Moss et al. 2010). However, here I present only the results for RCP 8.5, which assumes humanity’s greenhouse gas emissions will continue to increase at a rate similar to the present.
Nowcasting Results
Using climate variables (e.g., temperature and precipitation) alone, the final elastic net regression model explained 80% of the variation in annual acres burned in YNP. The regression also correctly predicted the rank of the historical fire years in terms of annual acres burned, i.e., the top five largest fire-years predicted by the model match the actual fire data. In descending order, the top fire years were 1988, 2016, 2003, 2007, and 2002.During the fire season, fire danger from this algorithm is reported daily, both as a fire danger rating number (ranging from -6 representing least severe to 13 or most severe) and as the number of acres that could be expected to burn if a fire were left unchecked (figure 2). These daily reports are emailed to interested people and also appear on the website at http://www.ClimateAnalyzer.org/y_fire.
The predicted level of fire risk responds very rapidly to both increasing drought and precipitation events. In some cases, it can double or halve in a single day (figure 3). A video showing the daily progression of fire risk during a wet year (1993), a dry year (2016), and the most recent fire year can be viewed at https://youtu.be/vvpXiZ-UjNI.
Forecasting
Under the RCP 8.5 emissions scenario (figure 4), which is most similar to the currently observed growth in humanity’s greenhouse gas emissions, the median model forecast (dashed red line, figure 4) calls for the decades in the mid-21st century to routinely resemble the ten years which ended in 2016, which is historically second only to the 1988 fires in terms of severity. By the end of the 21st century, the median forecast calls for approximately 50% of the park to burn every decade, and the worst forecast calls for roughly 150% of the park burning every decade. The lowest forecast (bottom red line, figure 4) indicates that approximately 3% of the park will burn (about 65,000 acres; 26,315 ha,) each decade by the end of the century. These low forecasts were produced when the Centre National de Recherches Météorologiques Coupled Global Climate Model, version 5 (CNRM-CM5) future climate model was used as input for the regression. CNRM-CM5 forecasts that spring snow will persist nearly as late as it does now, producing relatively short fire seasons throughout the 21st century (see Tercek and Rodman 2016 for more discussion).Implications
The fire forecasting tool presented here uses a single set of methods to link real-time “nowcasts” of YNP’s fire danger to broad-brush forecasts decades in the future. Corroborating other authors (e.g., Romme and Despain 1989, McKenzie and Littell 2017) who found YNP’s fire regime to be more climate-limited than fuel-limited, I found that about 80% of the variability in annual acres burned can be explained by drought-related variables derived from a water balance model. The results of this tool, which are much less time consuming and resource-intensive to compute than the complex models cited in the beginning of this article, are now available to fire managers and the public as automatically-generated, daily, fire nowcasts on www.ClimateAnalyzer.org.How much precision can be ascribed to the nowcasts (figure 2)? In 2017, for example, there were no significant fires in the park, even though the fire predictions called for more than 30,000 acres of expected burn for several days during the end of the season (figure 3). Clearly, the size of the fires that occurs in the real world depends on more than just drought, as measured by the elastic net regression. The 20% of the variability not included in the model does indeed include fuel availability (see above), fire suppression efforts, and other factors such as the presence of lightning when drought conditions are most favorable to start a fire. A fire needs to start in the right place and be left alone long enough to get established before any of the climate factors in my model apply. Even if lightning did strike in a favorable place and time, for example on September 12, 2017, it would not be realistic to expect exactly 35,799 acres to burn (figure 3). No forecasting algorithm is that accurate.
The best interpretation of the nowcasts would be to focus on powers of 10, i.e., the large scale divisions on the y-axis of figure 2. For example, the green dot in figure 2 indicates the forecast for August 26, 1993, calls for 2 acres to burn, but a more realistic reading would be to say the climate potential indicates less than 10 acres are expected to burn. Similarly, the red dot in figure 2 (August 26, 2016) indicates 100,000 - 1,000,000 are expected to burn, while the blue dot (August 26, 2017) indicates 1,000 - 10,000 acres. I base this “power-of-10” interpretation on the fact that the number of acres reported in the nowcasts (figure 4) are actually the exponentiated (i.e., “un-log-transformed”) results of a regression that varies on a logarithmic scale, as well as the realization that a broad power-of-10 interpretation is probably all that matters in a practical, decision-making context. When fire danger reaches the 10,000-1,000,000 acre range, as it did in 2016, pretty much everything burns, including young forests that were newly regenerated from 1988. Fire danger ratings lower in the power-of-10 scales merit proportionately less management concern.
The most likely long-term fire forecasts are the upper two red lines in figure 4. I base this suggestion on two facts. First, the RCP 8.5 results shown here are based on greenhouse gas emission increases that are most similar to those currently observed, while the RCP 4.5 results (not shown in this summary article) are based on the assumption that humanity quickly develops a much smaller carbon footprint (Moss et al. 2010). Second, the lower red line (least burn forecast) is based on a future climate model that assumes most future snow loss in the Yellowstone area will occur in the fall rather than the spring, an assumption that is not validated by historical climate data (Tercek and Rodman 2016).
How should we respond to these long-term fire forecasts? If the upper two forecasts (figure 4) are indeed the most accurate, then the climate during the end of the 21st century will be dry enough to support fires that burn 50%-150% of YNP every decade. One response would be to agree with Schoennegal et al. (2017) and conclude that frequent, large-scale fires are an unstoppable part of our new reality:
. . .wildfire policy and management require a new paradigm that hinges on the critical need to adapt to inevitably more fire in the west in the coming decades. Policy and management approaches to wildfire have focused primarily on resisting wildfire through fire suppression and on protecting forests through fuels reduction on federal lands. However, these approaches are inadequate to rectify past management practices or to address a new era of heightened wildfire activity in the west.
This acceptance might be forced on us for economic reasons. Fighting fires is already very costly, and it is unlikely we will be able to scale up our efforts to meet the forecast increases. During the 2017 fire season, fires in Montana burned roughly 1.2 million acres and cost $1.5 million per day to fight (Smith 2017). Fire-fighting nationwide consumes more than half of the U.S. Forest Service’s budget, or approximately $2 billion per year (Worby 2017).
A second response to the forecast increases in fire would be to make every effort to preserve at least some of YNP’s ancient conifer forests to buy time until a better solution is invented (the forests along the Soda Butte Creek drainage are a good example). Some climate scientists and ecologists argue that humanity’s greenhouse gas emissions have already exceeded safe levels, and that massive, industrial scale removal of carbon dioxide from our atmosphere is the only hope of avoiding “unacceptable” losses of life, habitat, and infrastructure (Kolbert 2017). If such carbon removal technology is eventually implemented, then efforts expended now to save at least some of YNP’s defining greenness will not be in vain. Some people will wish to enjoy the park as we know it for as long as possible; however, if massively-scalable carbon removal technology is in fact invented, we will regret not having saved forests that would be newly viable.
Whichever response is adopted, the tool presented here will be useful. Under either strategy, we can choose to let fires burn more often when conditions are less dangerous. If we accept that massive fires are inevitable and ultimately cannot be prevented, it might still be prudent to eliminate let-burn policies and put out all fire starts immediately when conditions indicate there is an unusually high risk of spread, making it impossible to control the fire’s direction. If instead we choose to preserve some old forests, then it might make sense to implement a strategy that incorporates controlled burns in surrounding habitats during times when the tool indicates fire risk is low.
Literature Cited
Abatzoglou, J., and A. Williams. 2016. Impact of anthropogenic climate change on wildfire across western US forests. Proceedings of the National Academy of Sciences 113:11770-11775.
Alexander, M.E. 1990. Computer calculation of the Keetch-Byram Drought Index – programmers beware! Fire Management Notes 51:23- 25.
Bowles, M. 2015. Machine learning in python: essential techniques. John Wiley and Sons, Indianapolis Indiana, USA.
Clark, J.A., R.A. Loehman, and R.E. Keane. 2017. Climate changes and wildfire alter vegetation of Yellowstone but forest cover persists. Ecosphere 8(1): e01636.
Despain, D.G. 1990. Yellowstone vegetation. Roberts Rinehart Publishers, Boulder, Colorado, USA.
Hansen, A., N. Piekielek, T. Chang, and L. Phillips. 2015. Changing climate suitability for forests in Yellowstone and the Rocky Mountains. Yellowstone Science 23:36-43.
Keetch, J.J., and G.M. Byram. 1968. A drought index for forest fire control. Research Paper SE-38. U.S. Department of Agriculture, Forest Service, Southeastern Forest Experiment Station, Asheville, North Carolina, USA.
Kolbert, E. 2017. Can carbon-dioxide removal save the world? November 20, 2017. The New Yorker, New York, New York, USA.
Logan, J.A., W.W. Macfarlane, and L. Wilcox. 2010. Whitebark pine vulnerability to climate-driven mountain pine beetle disturbance in the Greater Yellowstone Ecosystem. Ecological Applications 20: 895-902.
Lutz, J.A., J.W. van Wagtendonk, and J.F. Franklin. 2010. Climatic water deficit, tree species ranges, and climate change in Yosemite National Park. Journal of Biogeography 37:936-950.
McKenzie, D., and J.S. Littell. 2017. Climate change and the eco-hydrology of fire: will area burned increase in a warming western USA? Ecological Applications 27:26-36.
Millspaugh, S.H., C. Whitlock, and P.J. Bartlein. 2000. Variations in fire frequency and climate over the past 17,000 yr in central Yellowstone National Park. Geology 28:211-214.
Mitton, J.B., and S.M. Ferrenberg. 2012. Mountain pine beetle develops an unprecedented summer generation in response to climate warming. The American Naturalist 179:E163-E171.
Moss, R.H., J.A. Edmonds, K.A. Hibbard, M. R. Manning, S.K. Rose, D.P. van Vuuren, T.R. Carter, S. Emori, M. Kainuma, T. Kram, G.A. Meehl, J.F.B. Mitchell, N. Nakicenovi, K. Riahi, S.J. Smith, R.J. Stouffer, A.M. Thomson, J.P. Weyant, and T.J. Wilbanks. 2010. The next generation of scenarios for climate change research and assessment. Nature 463:747-756.
Romme, W.H, and D.G. Despain. 1989. Historical perspective on the Yellowstone fires of 1988. Bioscience 39:695-698.
Rupp D., J. Abatzoglou, K. Hegewisch, and P. Mote. 2013. Evaluation of CMIP5 20th century climate simulations for the Pacific Northwest USA. Journal of Geophysical Research: Atmospheres 118: 10884-10906.
Schoennagel, T., J.K. Balch, H. Brenkert-Smith, P.E. Dennison, B.J. Harvey, M.A. Krawchuk, N. Mietkiewicz, P. Morgan, M.A. Moritz, R. Rasker, M.G. Turner, and C. Whitlock. 2017. Adapt to more wildfire in western North American forests and climate changes.
Proceedings of the National Academy of Sciences 114:4582-4590.
Smith, A. 2017. Montana’s tough summer. December 11, 2017, page 9. High Country News, Paonia, Colorado, USA.
Tercek M., and A. Rodman. 2016. Forecasts of 21st century snowpack and implications for snowmobile and snowcoach use in Yellowstone National Park. PLoS ONE 11(7):e0159218.
Thornthwaite, C.W., and J.R. Mather. 1955. The water balance. Publications in Climatology 8(1).
Thrasher B., J. Xiong, W. Wang, F. Melton, A. Michaelis, and R. Nemani. 2013. Downscaled climate projections suitable for resource management in the U.S. Eos, Transactions American Geophysical Union 94:321-323.
Westerling, A.L, H.G. Hildago, D.R. Cayan, and T.W. Swetnam. 2006. Warming and earlier spring increase western US forest wildfire activity. Science 313:940-943.
Westerling, A.L., M.G. Turner, E.A. Smithwick, W.H. Romme, and M.G. Ryan. 2011. Continued warming could transform greater Yellowstone fire regimes by mid-21st century. Proceedings of the National Academy of Sciences 108:13165-13170.
Westerling, A.L 2016. Increasing western US forest wildfire activity: sensitivity to changes in the timing of spring. Philosophical Transactions of the Royal Society B 371:20150178.
Worby, R. 2017. Proper fire funding continues to elude Congress. December 11, 2017, page 6. High Country News, Paonia, Colorado, USA.
Zou, H., and T. Hastle. 2005. Regularization and variable selection via the elastic net. Journal of the Royal Statistical Society B 67:301-320.
Mike Tercek has been living and playing in Yellowstone for nearly three decades. He got his PhD studying plants endemic to the park’s thermal areas, but most of his work now revolves around data analysis and writing computer code. He is the founder of Walking Shadow Ecology, based in Gardiner, Montana.
Last updated: September 16, 2019