Last updated: August 25, 2017
Article
Landscape response to climate change and its role in infrastructure protection and management at Mount Rainier National Park
In November 2006 an intense storm dropped 45 cm (17.9 in) of rain over 36 hours at Mount Rainier National Park, Washington. The record floods from this event caused the park to be closed for six months, destroying roads and trails, damaging utilities, and cutting off access to park campgrounds. The region overall has seen increasing flood damage: six of the largest storms on record have occurred in the last 25 years (Parzybok et al. 2009). Flooding at Mount Rainier is not uncommon, but the November 2006 flood was by far the most destructive in park history and its effects are still felt today. Though one might conclude that the 2006 flood was an anomalous event in the park’s history, recent trends in flooding, aggradation (excessive sediment accumulation in riverbeds), debris flows, glacial recession, and warming temperatures are consistent with effects of climate change.
Because of steep topography, Mount Rainier hosts significant development in valley bottoms near rivers. Large portions of the mountain’s infrastructure are built in the rustic style of architecture from the early 1900s, and make up the park’s designated National Historic Landmark District (NHLD), the highest level of cultural resource protection. Much of this development occurred before recognition of hazards associated with building near rivers. For example, the Carbon River Road on the northwest side of the park was built along the grade of the river. Repeated flooding in the 1990s and subsequent destructive floods in 2006 and 2008 have damaged significant portions of the road, cutting off access to the northwestern portion of the park. The Longmire Complex, which is one of
the main developed areas on the west side of the park, is located adjacent to the Nisqually River. This river is up to 30 feet in elevation higher than nearby roads and park buildings, which are only protected by a small levee ( Fig. 1). Park staff must determine how best to manage infrastructure in peril, including areas that have been designated as NHLD features.
A changing landscape
Steep moraines and debris rich glaciers continually provide sediment to Mount Rainier’s rivers, causing them to develop into an intricate system of complex braided channels. The riverbeds, on average, are rising by natural sedimentation and aggradation processes. For the samesize
storms, the flood potential is ever increasing since the capacity of the river channels is reduced as they fill in with sediment (Beason 2007; Riedel 1997). Park rivers aggraded, on average, 0.3 m (12 in) per
decade from 1997 to 2006. The rate has increased recently to an average of 1.4 m (57 in) per decade on the Nisqually River from 2005 to 2010—and, following debris flow deposition, up to 1.8 m (6 ft) in a single event (Beason 2007).
Climate models run by the University of Washington’s Climate Impact Group indicate that more winter precipitation will fall as rain rather than snow by 2100. In mid elevation ranges, this change will result in less snow accumulation, higher winter and lower summer streamflows, earlier snowmelt, and earlier peak streamflow (Hamlet et al. 2010; Snover et al. 2003). Understanding variability in climate and the effects on river hydrology is extremely important for assessing future conditions for parks.
Recent findings concerning rivers at Mount Rainier support predictions of changing precipitation patterns and hydrology. Rainfall events exceeding 8 cm (3 in) in 24 hours had an additional 2.5 cm (1 in) of precipitation between 1976 and 2006 (Abbe et al. 2008). The magnitude of the 100year flood has also changed. For example, on the Nisqually River, these levels have increased from 387 m³/s (13,670 ft³/s) in 1972 to 626 m³/s (22,111 ft³/s) in 2009 ( Fig 2). Stated another way, a flood of 387 m³/s (13,670 ft³/s) had a 1% probability of occurring in the past, but now has an 8% chance of occurring. The 100year event in 1972 now has a recurrence interval closer to that of a 12year event. This increase in peak flows challenges the ability to understand and reliably predict the frequency and magnitude of large floods.
Glacier dynamics
Major rivers at Mount Rainier National Park are glacier sourced, and proglacial valleys (valleys previously carved out by the glaciers when they were advancing) control their forms and patterns. Glaciers are a critical natural resource at Mount Rainier and an important vital sign for biophysical monitoring in the North Coast and Cascades Network. Mount Rainier has more glacial ice in its 25 named glaciers and innumerable perennial snowfields than any other peak in the contiguous United States—more, in fact, than all the other Cascade Range volcanoes combined.
Studies of glaciers on Mount Rainier have shown an overall decrease in volume. Total ice volume was estimated to be 5.62 km³ (1.35 mi³) in 1913 and to have decreased by 22.7% (to 4.34 km³ or 1.04 mi³) by 1971, at a loss of 0.39% per year (Nylen 2001). In 1981, the total glacier volume was measured at
4.42 km³ (1.06 mi³) (Driedger and Kennard 1986). From 1971 to 1994, the rate of glacial volume loss decreased, averaging 0.13% per year with a 3.1% loss during that interval (Nylen 2001). However, Sisson et al. (2011) estimated 14% glacial ice loss between 1970 and 2008, an average of 0.37% per year, which indicates that the rate of loss sped up considerably between 1994 and 2008. Glacier volume in 2008 was estimated at approximately 3.82 km³ (0.92 mi³) (Sisson et al. 2011). The rate of glacier loss appears to have shot up in recent years: new surveys on several glaciers on Mount Rainier indicate that total glacial volume has potentially decreased by as much as 18% from 2003 to 2009 at an average rate of 3% per year, almost 10 times any of the historical rates (NPS, J. Riedel, geologist, personal communication, 1 March 2009).
Since 1931, surveys of the lower Nisqually Glacier have been conducted to determine changes in elevation of the ice surface, making it one of the longest running glacier monitoring programs in the Western Hemisphere. These surveys show how the Nisqually responds to changes in snow accumulation. When higher than normal snowfall builds up in the accumulation zone of the glacier, a bulge forms, which can be tracked moving down the glacier. These bulges, called kinematic waves, can exceed 24 m (80 ft) in height, and move down glacier at speeds up to six times faster than the ice itself. When the wave reaches the terminus, the glacier usually advances dramatically. Four kinematic waves have been observed since the project began, three of which have resulted in glacier advances. The fourth wave, expected to reach the terminus in 2005, was accompanied by glacial retreat as glacial melt overwhelmed ice advance.
There are also concerns about stagnating ice on Mount Rainier. Average ice velocities on the Nisqually Glacier were previously measured at approximately 200 mm/day (8 in) (Hodge 1974). However, recent measurements by park staff show the movement is more like 200 mm per year (NPS, S. Lofgren, lead climbing ranger, personal communication, 3 November 2009). Debris rich stagnant glacier ice has been linked to destructive glacier outburst floods at Mount Rainier in the past (Walder and Driedger 1994). Glacier loss is also linked to increased sediment availability, destructive debris flows, and outburst floods. Debris flows are fast moving liquefied landslides of soil, rocks, trees, and anything else in their paths. Mount Rainier has recently experienced many debris flows, and increases are predicted as glaciers continue their rapid retreat. Since 2001, there have been at least six debris flows in three watersheds that have not had such flows since the park was founded more than a century ago.
During the 2005 and 2006 floods alone, 10 debris flows occurred in two days, resulting in extensive infrastructure damage. All debris flows began in areas that were deglaciated since 1913 (Copeland 2009).
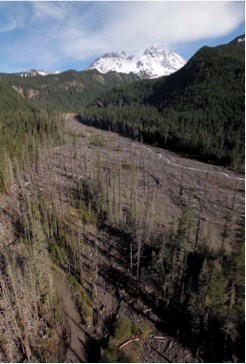
Debris flow statistics alone do not prove a recent increase in debris flow frequency; the record is incomplete. Coupled with extreme landscape response (fig. 3, above), however, the record strongly suggests an increase. Future research will use dendrochronology to extend the debris flow record and reveal whether debris flow frequency has increased as suspected. Most recent debris flows have occurred in warm autumn storms, when perennial snow cover is minimal or absent—a condition future climate change is expected to produce more frequently.
Hazards
Sediment mobilized by debris flows is transported to lower gradient river valleys. The sheer volume of material in a debris flow can overwhelm the river’s transport capacity and choke off a river valley, causing it to accumulate a vast amount of sediment. For example, the confluence of the Nisqually River and Van Trump Creek has aggraded 11 m (36 ft) since 1910 because of debris flow activity (Beason 2007). Decreased glacial volume and area may lead to increasing sediment supply from steep, unstable moraines. Ground that was previously overtopped with glacial ice in steep portions of the mountain provides a readily available sediment source for debris flows; this is occurring in the vicinity of the Van Trump Glaciers and has been the source of repeated debris flows (Copeland 2009). Additionally, periglacial ice (ice near glacial margins) may act as a structural support for loose debris, and as permafrost and periglacial ice sources are lost, so too is this support. All these mechanisms will likely lead to increasing sediment production. In turn, more sediment will be provided to already choked river channels. Over time the conveyance capacity, or amount of water a channel can transport (or carry), will decrease. Therefore, for the same size floods, the inundated area of the floodplain will increase and may affect riparian habitat and historical infrastructure. Floodplains are also threatened by the prospect of warmer and more intense storms, which could dramatically change river hydrology. Avulsions, or rapid migrations of a river channel from its previous course, will be more likely and have been documented on the White River and Tahoma Creek (Cardno ENTRIX 2010; Walder and Driedger 1994).
An uncertain future
Mount Rainier is a dynamic geologic landscape experiencing major changes; these changes are consistent with and likely to increase in response to regional climate change. Management complexities and knowledge gained by scientists at Mount Rainier can be applied to other places in the world. While it is impossible to control natural forces, significant progress is being made in understanding these phenomena. National Park Service scientists, along with researchers from Oregon State University, Portland State University, the University of Washington, and the U.S. Geological Survey, are scrutinizing the in park and downstream response of the landscape and what it means for the future. Such studies suggest that aggradation is occurring in and causing problems for rivers emanating from the mountain; evidence is that these issues are not confined to the park boundary (Czuba et al. 2010). Results from current and future studies will add to a growing body of science that investigates landscape response to climate change and its role in management of
resources and overall visitor and employee protection. Mountains and rivers are dynamic systems, and their change is natural. The violent, volatile events brought on by climate change, however, pose a wide variety of serious challenges to ecosystems and infrastructure. Protecting infrastructure often results in the tradeoff of damaging ecosystem components, functions, and processes. Over time, agencies charged with overseeing natural places, landscapes, and wildlife will need to evolve and adapt to these drastic and sweeping changes.
References
Abbe, T., P. Kennard, J. Park, and S. Beason. 2008. Alluvial landscape response to climate change in glacial rivers and the implications to transportation infrastructure. Presentation at 2008 National Hydraulic Engineering Conference, 26–29 August, Portland, Maine, USA.
Beason, S. R. 2007, The environmental implications of aggradation in major braided rivers at Mount Rainier National Park, Washington. Thesis. University of Northern Iowa, Cedar Falls, Iowa, USA.
Cardno ENTRIX Environmental Consultants. 2010. White River geomorphic reach analysis: Phase 1 report, Mount Rainier National Park. Cardno ENTRIX Environmental Consultants, Seattle, Washington, USA.
Copeland, E. A. 2009. Recent periglacial debris flows from Mount Rainier, Washington. Thesis. Oregon State University, Corvallis, Oregon, USA.
Czuba, J. A., C. R. Czuba, C. S. Magirl, and F. D. Voss. 2010. Channel-conveyance capacity, channel change, and sediment transport in the Lower Puyallup, White and Carbon rivers, western Washington. USGS Scientific Investigations Report 2010-5240. U.S. Geological Survey, Tacoma, Washington, USA.
Driedger, C. L., and P. M. Kennard. 1986. Ice volumes on Cascade volcanoes: Mount Rainier, Mount Hood, Three Sisters and Mount Shasta. USGS Professional Paper 1365. U.S. Geological Survey, Vancouver, Washington, USA.
Hamlet, A. F., P. Carrasco, J. Deems, M. M. Elsner, T. Kamstra, C. Lee, S.-Y. Lee, G. Mauger, E.P. Salathe, I. Tohver, and L. Whitely Binder. 2010. Final project report for the Columbia Basin Climate Change Scenarios Project. Accessed 3 March 2011 at http://cses.washington.edu/db/pubs/allpubs.shtml http://cses.washington.edu/db/pubs/allpubs.shtml.
Hodge, S. M. 1974. Variations in the sliding of a temperate glacier. Journal of Glaciology 13(69):349–369.
Nylen, T. H. 2001. Spatial and temporal variations of glaciers (1913–1994) on Mt. Rainier and the relation with climate. Thesis. Portland State University, Portland, Oregon, USA.
Parzybok, T. W., D. M. Hultstrand, E. M. Tomlinson, and W. D. Kappel. 2009. Real-time depth-area-duration analysis for EAPs and flood warning systems. Presentation at ADSDO Annual Dam Safety Conference, 27 September–1 October, Hollywood, Florida, USA.
Riedel, J. L. 1997, Geologic hazards and floodplain management. Mount Rainier General Management Plan. Unpublished internal document. National Park Service, Ashford, Washington, USA.
Sisson, T. W., J. E. Robinson, and D. D. Swinney. 2011. Whole-edifice ice volume change 1970–2007/8 at Mount Rainier Washington based on LiDAR surveying. Geology 39(7):639–642.
Snover, A. K., A. F. Hamlet, and D. P. Lettenmaier. 2003. Climate change scenarios for water planning studies: Pilot applications in the Pacific Northwest. Bulletin of the American Meteorological Society 84(11):1513–1518.
Walder, J. S., and C. L. Driedger. 1994. Geomorphic change caused by outburst floods and debris flows at Mount Rainier, Washington, with emphasis on Tahoma Creek valley. U.S. Geological Survey Water Resources Investigations Report 93-4093. U.S. Geological Survey, Vancouver, Washington, USA.