Last updated: January 29, 2018
Article
Monitoring River Systems and Fluvial Landforms
Department of Geosciences and Natural Resources, Western Carolina University, Cullowhee, North Carolina 28723, USA
Dru Germanoski
Department of Geology and Environmental Geosciences, Lafayette College, Easton, Pennsylvania 18042, USA
Nicholas E. Allmendinger
Department of Watershed Sciences, Utah State University, Uintah Regional Campus, Vernal, Utah 84078-4126, USA
Purpose and Scope
The purpose of this chapter is to provide an overview of river and stream dynamics, describe possible stressors that may lead to channel instability, and provide guidelines and methods for monitoring streams and rivers. Because there are great differences in budget, staffing, and management needs and objectives between managed lands, we provide procedures for three levels of monitoring protocols. The three levels progress from low-budget methods where minimal expertise in fluvial geomorphology is required, to higher-level methodologies requiring greater expertise, a larger budget, and more time. We recognize that personnel range from people having no formal training in fluvial geomorphology to people holding graduate degrees in the field. In the past decade, there has been increased emphasis on stream water quality, monitoring, and restoration from government and nongovernment associations. With this, people from a wide variety of backgrounds have become involved in monitoring and sampling of streams. While, indeed, many methods for sampling and monitoring are simple, the design of a study and interpretation of data are not simple—they require a sophisticated understanding of stream systems. Improper study design and interpretation has led to, in many cases, the collection of useless data, or worse, the implementation of land use plans or stream restoration projects that made problems worse. Because of this, we have put considerable emphasis in the overview of fluvial geomorphology to describe how stream processes relate to channel form and the dynamics of these complex, highly interconnected systems. Understanding how a stream works, how it relates to other systems (natural and artificial), and how it relates to the entire watershed defines the core of knowledge necessary for designing a successful monitoring plan and interpreting the data properly.To the extent possible, in this short treatment of the subject, we aim to provide a description of methodologies that are useful to most readers. Good descriptions of methods for monitoring fluvial systems are increasingly abundant because of the environmental emphasis on stream systems and because of the on-going effort of many agencies, mostly government, to make methods available on their Web sites. More experienced individuals would be well served to read this chapter and then use a more comprehensive, detailed coverage, such as Kondolf and Piégay (2003).
This chapter focuses on monitoring fluvial systems and does not attempt to provide guidelines for channel restoration. A philosophical statement and guidelines for river restoration are given in Doyle et al. (1999) and by the Applied Fluvial Geomorphology Ad Hoc Committee of the Geological Society of America Quaternary Geology and Geomorphology Division (2004). Although short courses in river restoration are available (Rosgen, 1996), there is growing body of compelling literature and direct evidence that suggests that the level of expertise gained from a short course is not sufficient preparation to guide restoration efforts (Kondolf and Micheli, 1995; Miller and Ritter, 1996; Juracek and Fitzpatrick, 2003; Kochel et al., 2005). We strongly recommend that land managers consult experienced people holding graduate degrees in fluvial geomorphology for restoration efforts and complex problems.
BASICS OF FLUVIAL GEOMORPHOGY
Systems ApproachWhen evaluating rivers or streams, the natural tendency is to focus on a segment, or “reach” of the channel. Typically, the segment size is determined by the needs and scope of the project. With respect to a national park, the segment might be dictated by the park boundaries. Whereas the reach-specific perspective may appear to be a logical approach, it will invariably prove to be insufficient for most purposes. Any segment of a river must be recognized as being a part of an integrated system, and the characteristics and dynamics of a segment of interest will be significantly affected by circumstances prevailing in the drainage basin upstream of the reach, and may also be affected by processes and events occurring downstream (Schumm, 1977). Therefore, any section of stream should be evaluated within the context of its position in a drainage basin or watershed, regardless of whether the upper basin is within or extends outside of park boundaries. The upstream drainage basin delivers sediment and water to the channel, and significant changes in the hydrology or sediment delivery from the watershed have the potential to change the equilibrium conditions in the channel (Fig. 1). Likewise, instability originating in the downstream segments of a system has the potential to migrate upsystem and destabilize a section of channel far removed from the site of initial disturbance (Schumm, 1977). This instability is commonly reflected by a knickpoint, which is a sharp break in slope of the longitudinal profile of a stream. In every case, it is important to view any segment of river within the framework of a fluvial system (Schumm, 1977; Petts and Amoros, 1996).
Drainage Basin Inputs
The drainage basin or watershed is a zone of production, where precipitation accumulates to be delivered to streams and rivers, and sediment is produced by landscape erosion (Schumm, 1977). When precipitation falls on a landscape, some portion of the water seeps into the ground and makes its way to the drainage network as shallow subsurface throughflow or as slow flowing groundwater. Water that does not infiltrate into the subsurface flows across the surface and moves rapidly to the drainage network as direct runoff. Because flow rates vary with pathways, the hydrology of the stream network is directly related to the relative percentage of water following various flow paths from the landscape to the drainage channels. Flow path depends on a number of drainage basin and climatic characteristics such as the infiltration capacity of the soil and bedrock, topography, vegetation, seasonal effects (frozen ground, snow cover, and foliage), variation in precipitation intensity through time, and land use (Burt, 1992). Each drainage basin will have an annual hydrologic regime that results from the integration of all of these variables.
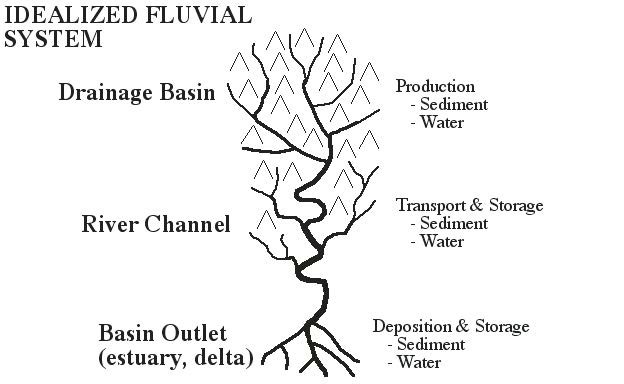
Rivers are dynamic landscape elements whose primary functions are to drain the landscape and transport sediment. Channel morphology (the cross-sectional, plan view and longitudinal configuration of a channel) develops a form that is adjusted to the regional topographic gradient, long-term average hydrologic regime, and sediment load of the drainage basin. In turn, invertebrates, fish, riparian vegetation (vegetation growing along the banks or on the floodplain), and wildlife adjust to the channel form and hydrologic and sediment transport regimes (Petts and Amoros, 1996). The entire system, physical and biological, develops an equilibrium condition in response to external controlling factors such as tectonics, geology, soils, climate, and land use (Schumm, 1977).
Typical, everyday (non-storm) flow does little to modify channel form, but, the question then arises, what is the channel-forming discharge? Clearly it must be a discharge that generates sufficient shear stress to move the material that comprises the bed and banks of the channel (Andrews and Nankervis, 1995). Although there are important exceptions, much data suggests that bankfull discharge (the discharge that fills a channel to capacity; the maximum discharge that a channel can convey without flooding) is the channel-forming discharge, and many channel morphological characteristics scale to bankfull discharge (Schumm, 1977; Leopold, 1994; Rosgen, 1996; Knighton, 1998). For most rivers, this discharge will occur once every two years or so, although tremendous variations exist from region to region and from river to river. For detailed discussions of the frequency of bankfull discharge and channel-forming discharge, see Williams (1978) and Castro and Jackson (2001).
Extreme rain or snowmelt runoff events are part of the normal dynamic, and although a major flood event may result in changes in the channel that may appear to represent episodes of instability and channel change, often these are temporary disturbances that rivers will recover from in subsequent months or years (Wolman and Gerson, 1978). Sediment transport also varies annually with discharge and supply from the watershed, and channel morphology and biological communities adjust to the long-term sediment transport of the channel. Sediment load is normally subdivided into three types. Material transported in solution or as dissolved chemical species in water is called dissolved load or solution load. Sediment transported in the water column suspended by turbulence is referred to as suspended load, whereas sediment transported by rolling, sliding, or bouncing along the bed is called bedload. In general, suspended load consists of fine-grained material such as clay and silt, and bed load normally consists of coarser material such as sand and gravel. However, the relationship between grain size and transport mode also depends on the flow energy of a given stream. Sand and even gravel-sized material can be transported as suspended load during high-energy transport events. Although there are important exceptions to the rule, suspended load is typically controlled by the production and delivery rates from the watershed, whereas bed load transport is more closely related to flow energy. For more detailed discussions of sediment transport and various methods of classifying sediment in transport see Richards (1982), Knighton (1998), or Bridge (2003).
River Channel Pattern and Morphology
Basic Controls
Channel morphology is influenced by the complex interplay among regional geology, climate, topographic gradient, river history, drainage basin hydrology, and sediment load. Although the interactions among these variables can be complex, most data suggest that drainage basin hydrology and channel discharge primarily determine the size of the channel, whereas the caliber and quantity of the sediment load determine channel pattern and cross-sectional morphology (Church, 1992). When describing or evaluating river channel patterns or channel morphology, it may be important to separate small headwater streams from large streams and rivers and to separate bedrock channels from alluvial channels. Bedrock channels change only over very long time frames, and for most short-term monitoring programs, it is reasonable to assume that changes in channel form in bedrock channels will be negligible. Therefore, the discussion that follows focuses on alluvial channels. Small headwater channels often exhibit unique characteristics; thus, some classification schemes developed for large rivers do not transfer directly to small channels. The classification schemes presented here loosely follow the approach presented by Church (1992).
Small and Intermediate Size Channels, and Incised Channels and Arroyos
Small and intermediate size channels. Small and intermediate size channels have dimensions that are scaled close to the size of bed material. Consequently, individual particles and accumulations of particles into bars or localized sediment deposits can have a significant influence on flow dynamics and channel morphology. Small channels, as defined by Church (1992), have flow depths that are closely scaled to large particles in the channel, such that particle diameter to flow-depth ratios are on the order of 0.1–1.0. In most cases, small channels are floored with cobbles (>64 mm) and boulders (>256 mm), and have steep gradients ranging from 2° to 20° (Church, 1992). Small streams, floored by coarse material and flowing down steep slopes, typically have a step-pool morphology (Grant et al., 1990), where the steps are formed of clusters of cobbles or boulders, large woody debris (Keller and Swanson, 1979), or even bedrock (Wohl and Grodek, 1994); the pools are flatter segments underlain by finer-grained material (Figs. 2 and 3F). Step-pool spacings are scaled to channel width; spacing between successive steps or successive pools typically ranges from 2 to 4 times channel width (Chin, 1989; Grant et al., 1990) and spacing is also inversely proportional to channel gradient (Whitaker, 1987; Grant et al., 1990; Abrahams et al., 1995). Measurements and observations in the field and in flumes, along with theoretical arguments, make it clear that steppool sequences represent equilibrium in high-gradient, coarsebed streams (Davies and Sutherland, 1980; Grant et al., 1990; Abrahams et al., 1995). Grant et al. (1990) suggest that particles that comprise the steps are typically mobilized only by low frequency, high magnitude discharge events with recurrence intervals of 50 years or more.
Church (1992) defines intermediate-size channels as those having water surface widths that are much greater than bed material particle diameters, but these channels are small enough that a large portion of their cross-sections may be blocked by bars (sediment accumulated to form discrete channel scale entities) or accumulations of large woody debris. Church (1992) suggests that in forested regions, intermediate scale channels would have widths less than ~20–30 m. In sand and gravel bed channels of this scale, the channel morphology is dominated by pool-riffle sequences, where pool-pool or riffle-riffle spacings are typically 5–7 times the channel width (Keller and Melhorn, 1978). Pools are often located adjacent to alternate bars in straight channel segments or adjacent to point bars in sinuous channels (Fig. 2). In forested regions, pools are often associated with accumulations of large woody debris (Keller and Swanson, 1979). Sand and gravel bed channels of this type are often dynamic, and lateral migration of the channel and redevelopment of pool-riffle locations may represent normal equilibrium behavior.
Incised channels and arroyos. Incised channels (channels that have downcut significantly below the valley floor giving them steep banks and low width:depth ratios) range from small gullies meters wide and meters deep, to entrenched channels and arroyos of tens of meters. These channels are typically formed in fine-grained material dominated by clay and silt-sized particles, although sand and gravel may be present in significant quantities. Arroyos and incised channels do not fit the dimensionless scaling criterion established by Church (1992), although in absolute dimensions they would be similar to the small and intermediate size channels described above. These channels are most common in areas with relatively low topographic gradients, and range from ephemeral to intermittent-flow channels in arid regions, to intermittent to perennial-flow channels in more humid regions (Schumm et al., 1984; Darby and Simon, 1999).
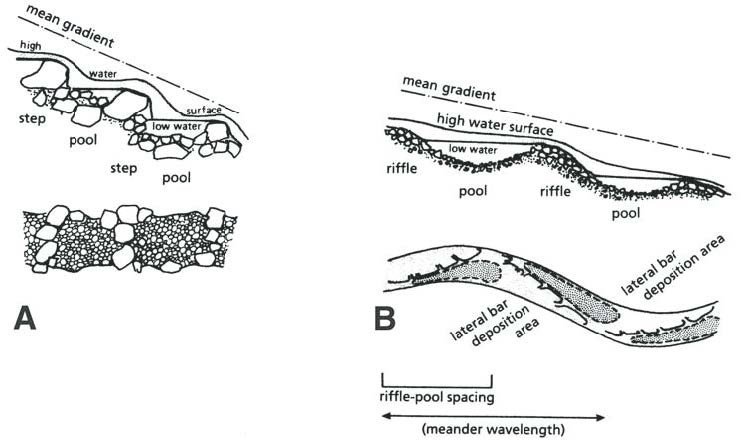
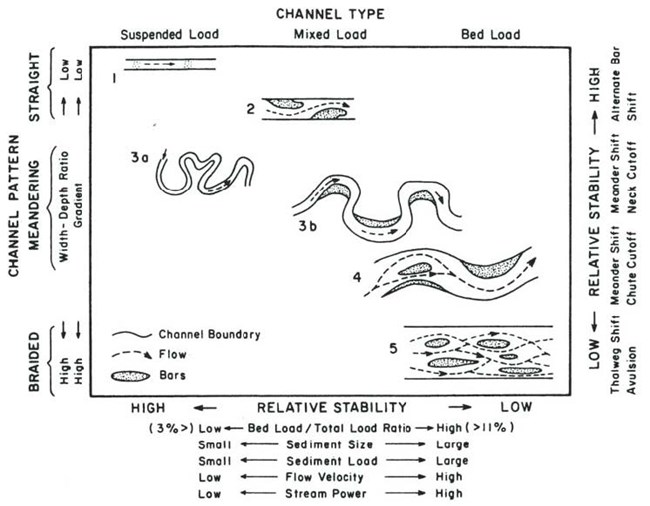
Large streams and rivers exhibit a wide range of morphologies, and a variety of classification schemes have been developed to describe channel patterns. Although classification boundaries are somewhat arbitrary because channel patterns grade along a spectrum, natural channels can be effectively categorized for descriptive purposes. We have adopted a scheme presented by Schumm (1981) and modified by Church (1992). This classification has the advantage of describing patterns in terms of controlling variables such as sediment load and stream power (the product of discharge and channel gradient), while incorporating other aspects of channel form and channel dynamics (Figs. 4 and 5). Rosgen (1996) has also developed a channel classification system (Fig. 6). Although we advocate use of the Schumm-Church approach, we present the Rosgen model because it has been used by some government agencies.
In the Schumm model, channels are separated into three sediment load categories: (1) suspended load-dominated, (2) mixed load, and (3) bedload-dominated (Fig. 4). Suspended load dominated channels range along a spectrum from straight single channels having low width depth ratios and low stream power (pattern 1, Fig. 4) to highly sinuous single-channels having low width-depth ratios and low stream power (pattern 3a, Figs. 3B and 4; stream type E in the Rosgen scheme, Fig. 6). Highly sinuous, suspended load–dominated channels are stable in form, but the channels migrate laterally and meander cutoffs (the process through which a stream cuts through the neck of a meander bend leaving the former channel as an abandoned channel segment) should be expected. Mixed load channels range from straight channels with alternate coarse-grained bars (pattern 2, Fig. 4) to sinuous channels with coarse-grained point bars (pattern 3b, Figures 3C and 4; stream type C in the Rosgen scheme, Fig. 6). Mixed load channels have higher stream power and higher width-depth ratios, and they transport larger quantities of coarse-grained sediment than suspended load–dominated channels. Sinuous mixed load channels have higher lateral migration rates than highly sinuous suspended load channels; erosion on the outside of meander bends and deposition on concave bank point bars are normal. These channels are typically characterized by well-defined pool-riffle sequences with spacing 5–7 times the channel width (Keller and Melhorn, 1978). Mixed load channels are also characterized by steeper gradients, high stream power, and higher sediment transport rates than suspended load channels. Bedload-dominated channels range from low sinuosity (the ratio of channel length to valley length) channels with point bars and a small number of mid-channel bars (pattern 4, Figures 3D and 4; stream type C or D in the Rosgen scheme, Fig. 6) to fully braided multi-channel rivers with many mid-channel bars (pattern 5, Figures 3E and 4; stream type D in the Rosgen scheme, Fig. 6). Bedload-dominated channels have high stream power, gradients, width-depth ratios, and sediment transport rates (Fig. 4). These channels are highly dynamic with mid-channel bars consumed and re-formed continuously and locally significant bank erosion. (Note the large number of trees deposited across the braid plain in Figure 3E; these trees became “organic load” as a result of bank erosion along the channel.)
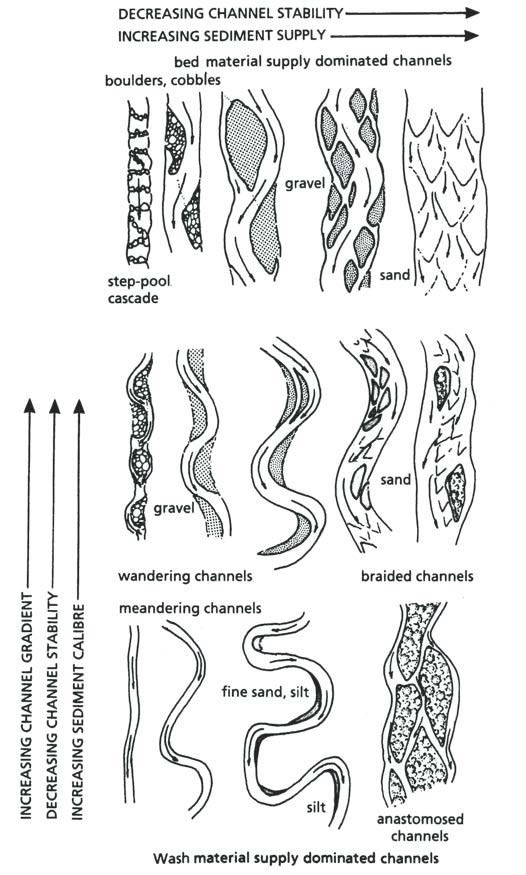
It is important to emphasize that each of the channel patterns described above may represent equilibrium morphologies that are adjusted to the prevailing sediment load and hydrology of any drainage basin. Pattern alone does not imply stability or instability. Likewise, any one of these patterns may represent a temporary morphology associated with an episode of channel instability. For example, some literature has suggested that braided channels are overloaded with sediment with the implication that the braided pattern is a non-equilibrium morphology. However, braided patterns could represent equilibrium, aggrading, or degrading conditions (Germanoski and Schumm, 1993). Instability can only be recognized when measurements reveal systematic changes in channel morphology or channel bed elevation.
The identification of unstable channels is an important, though not always easy, part of managing land. Lateral migration and channel avulsion (when a channel takes a new path) are not necessarily indicators of channel instability. Most natural channels are inherently dynamic; channels migrate laterally, and channel avulsion is a normal process. The degree to which individual channels change position is often characteristic of the particular morphology (Figs. 4 and 5). Instability is manifested by significant changes in channel bed elevation, changes in channel cross-sectional morphology (width:depth ratio or cross-sectional area), and/or channel pattern change. In some instances, unstable channels may maintain the same plan view pattern (the pattern or geometry of a channel when viewed from above) and the same width:depth ratio, but the channel may experience changes in cross-sectional area. In other instances, unstable channels may downcut or aggrade systematically while maintaining the same cross-sectional form and plan view morphology. Finally, unstable channels may change channel plan view form, channel bed elevation, or channel cross-sectional size in any possible combination. Typically, a channel is considered unstable if any of these changes are systematic.
A balance exists between sediment load and stream power, which is proportional to the product of discharge and channel slope. If sediment size or quantity delivered to a channel is reduced, it will tip the balance into a state of channel incision or degradation. Conversely, if sediment size and quantity delivered to a channel is increased, it may result in channel bed aggradation. In either case, degradation or aggradation may also be accompanied by changes in channel morphology. In a similar way, if stream power increases or decreases, a channel may degrade or aggrade and also may experience changes in channel morphology. Predictions of the possible morphological adjustments can be deduced from Figures 4 and 5.
VITAL SIGNS OF FLUVIAL SYSTEMS
Introduction
In this chapter, vital signs are a subset of characteristics of a larger fluvial system that can be monitored to provide information about the overall condition and trends of the system. The vital signs for fluvial systems are introduced here to provide a framework for understanding how fluvial systems respond to natural and anthropogenic stresses (see the next section). These vital signs are fundamental characteristics of fluvial systems; they should be of interest to those making management decisions related to fluvial geomorphology. They are also relevant to other natural resources being monitored in many managed lands (such as biology, water quality, and hydrology). Most importantly, data generated by monitoring these vital signs will permit evaluation of trends to assess and understand the impact of stresses on fluvial systems.
Six vital signs are described to monitor fluvial systems, three of which are directly related to the form of channel: longitudinal profile, planform, and cross-section. These vital signs are consistent with, though not identical in form, to those suggested by the Geoindicators Initiative (Berger, 1996; Osterkamp and Schumm, 1996). The fluvial vital signs are not independent of each other. As stated well by Osterkamp and Schumm (p. 97, 1996), “The processes in one part of the drainage basin cannot be separated from those in another part; thus, measurable change at one site often results in measurable change in others. In this sense, rivers and the valleys they drain are closely linked, and they must be treated as parts of a single, dynamic system.”
Vital Signs
1. Watershed Landscape
Definition. Natural and artificial characteristics of the land cover and surface materials throughout the entire watershed. Changes in vegetation, land use, surface geologic materials (soils, sediments, and rocks), slopes, and hydrology may be monitored to assess this vital sign.
Significance. Changes in land cover and materials may be caused by fires, volcanism, climatic change, logging, land use, roads, and other factors. Any change will directly affect the watershed hydrology and may affect any other part of the fluvial system, such as stream form, sediment transport, water quality, flood frequency, and the quality of stream habitat.
2. Hydrology
Definition. Frequency, magnitude, and duration of stream flow rates and their relationship to rainfall, snowmelt, groundwater, and vegetational patterns. Changes in stream hydrology may be monitored directly or through vegetational patterns. Paleohydrology studies may be used to better understand past hydrologic patterns and causal relationships.
Significance. Water drives fluvial systems and connects all parts of a watershed. It is also directly linked to climatic and biologic systems. Hydrologic patterns can react immediately to changes in the watershed; this is unlike other fluvial vital signs, which may take years to decades to respond to changes in forcing mechanisms.
3. Sediment transport
Definition. The rates, modes, sources, and types of materials transported by a stream. Changes in sediment transport may be linked to hydrologic or channel vital signs monitoring programs.
Significance. Sediment transport is very responsive to changes in the watershed, due to changes in both hydrology and physical characteristics. Sediment transport rates are directly related to turbidity and, consequently, are important to water quality.
4. Channel: Cross-section
Definition. The shape, position, and materials of a stream channel cross-section at a specific point. Stream cross-section geometry and characteristics may be monitored at key reaches of scientific or management concern.
Significance. Changes in a channel cross-section can provide readily quantifiable data to determine migration rates, high water marks, and areas of deposition and erosion. As with other channel vital signs, changes in cross-section may be caused by reach or watershed scale changes in hydrology or sediment budgets or natural stream dynamics.
5. Channel: Planform
Definition. The channel type, sinuosity, position, width, and morphology. Changes in channel planform characteristics, including depositional bar forms and materials, may be directly monitored or evaluated by examination of historic aerial photographs and maps.
Significance. Changes in the hydrologic or sediment budgets (the long-term average volume of water or sediment delivered to a segment of a channel), at the watershed or reach scale (a reach of a channel is a segment of a channel), will likely cause changes in channel form and structure. Any change in channel structure will likely cause changes in the quality of stream habitats.
6. Channel: Longitudinal Profile
Definition. The slope of the stream along its axis, including stream characteristics such as knickpoints, riffles, pools, and bed materials. Stream longitudinal characteristics and slope can be monitored from scales of a small reach to the entire stream length.
Significance. Changes in the watershed hydrologic or sediment budgets will likely cause changes in stream slope, through aggradation or degradation, and structure. The stream structure (e.g., riffle-pool spacing, bed materials) is directly linked to the quality of stream habitats. In some alluvial streams, knickpoints can migrate rapidly upstream and cause a decrease in the water table and shifts in types of riparian vegetation.
CHANGE IN FLUVIAL SYTEMS
Introduction
The purpose of this section is to discuss the primary stressors that are likely to affect fluvial systems and describe how the vital signs may respond to those stressors. Stressors, as used herein, are forcing mechanisms that can induce change in a fluvial system. These agents of change can be natural or artificial, be driven by forces within a watershed, such as logging, or driven by forces external to the watershed, such as climatic change, and may have a detrimental or beneficial impact on a fluvial system. Simply, stressors cause change in the fluvial systems that may result in instability.
Not all change, however, is due to stressors. Some change is constant in a fluvial system. For example, most naturally meandering streams have channels that migrate laterally. This change is not due to any stressor acting on the system, but merely reflects how the stream operates in a condition of dynamic equilibrium. Understanding the rate of this lateral migration may be of value to management of land even though it is not a response to a stressor. Alternatively, rates of lateral migration may increase significantly in response to a stressor, such as increased storm flow discharges due to altered hydrology or weakening of channel banks due to removal of riparian vegetation.
System Characteristics
Prior to a discussion of stressors and responses in fluvial systems, it is beneficial to review several system characteristics that are fundamental to performing studies of fluvial systems. Foremost, as discussed previously, stream systems are inherently interconnected, not just to fluvial components, but directly to vegetation, soils, water quality, hydrology, and humans. Streams have existed with wide ranges of variability for millions of years prior to any human influence; however, it is now difficult to imagine even one part of the fluvial system that has not been influenced by human actions. A common objective of fluvial studies is to determine the human influence on a stream, but in many cases the response of a stream cannot be singularly ascribed to human causes because of the inherent natural variability of these dynamic systems.
In geologically and climatically stable areas, most stream systems make predictable adjustments to accommodate common, natural variations in water and sediment inputs. Streams are nature’s pipes adjusted to the most efficient condition to transport water and sediment within a watershed (Ritter et al., 2002). Anything that changes the magnitude or spatial or temporal patterns of water and sediment inputs will cause the stream to adjust to those new conditions. In some cases, adjustments in the fluvial system may cause a threshold to be crossed and the stream will change to a new condition, for example, from a meandering to braided pattern in response to an increase in sediment load. The magnitude and the spatial and temporal patterns of response will be dictated not only by the changes in inputs, but by the sum of Earth’s history in that watershed, from conditions as recent as basin soil moisture conditions two days ago to the geologic framework (sediments, rocks, topography, drainage network) established over millions of years.
The intricacies of possible responses in a fluvial system to stressors are often complex and unpredictable; this characteristic is termed complexity or complex response (Schumm, 1991). Additional traits of the complexity of fluvial systems important to establishing and understanding cause-effect relationships are singularity, divergence, and convergence (Schumm, 1991). Singularity acknowledges that well-established generalizations may not be applicable for site-specifi c predictions because of unexplained (or unknown) variations at a site. For example, extensive fires in a watershed commonly lead to increases in storm-generated flow and sediment transport from the fire scarred landscape. A specific drainage basin, however, may show little impact from a fire if it does not receive a precipitation event large enough to move sediments prior to the reestablishment of vegetation (Germanoski et al., 2002). The terms convergence and divergence further describe the complexity in establishing cause-effect relationships. Convergence is the condition where different processes (causes or stressors) can result in the same response (effect). Divergence is the condition where the same stressor (cause) can yield different responses (effects). The example above can demonstrate convergence; an increased rate of lateral migration of a stream (the effect) may be due to decreased strength of channel banks caused by removal of vegetation or increased storm flow discharges. Likewise, two watersheds may respond differently (divergently) to a similar scale fire because one basin received a precipitation event large enough to mobilize sediments prior to the reestablishment of vegetation, while the other did not.
Stressors of Fluvial Systems
There are innumerable individual natural and anthropogenic stressors that can affect fluvial systems. Fortunately, individual stressors can be grouped into a handful of categories based on how and where they affect the processes or properties in fluvial systems (Table 1). At the most basic level, the stressor category altered hydrology includes any individual stressor that may affect pathway, rates, frequency, or location of water movement in a watershed. This change in hydrology may be due to specific stressors such as climatic change, forestry practices, or withdrawal of stream water for consumption. Similarly, any specific stressor that may affect the availability and transport of sediment falls in the stressor category of altered sediment budget.
While basin-scale stressors are limited to the two general categories above, an additional category of stressor is included for channel-scale stressors—altered channel. This category
includes individual stressors that directly affect the stream channel, such as dredging, trail crossings, channelization, and trampling of banks by grazing animals. In addition to the scale at which the stressor acts and the stressor category, stressors are also grouped by source area (Table 1). The source of many stresses is within the watershed, but others, such as climatic change, are due to outside changes.
The only thing completely predictable in a fluvial system is that change will occur. In response to a change in a stressor, the changes in a fluvial system may be direct or indirect, occur immediately or long after the initial change, may be large scale or small scale, may have an initial response that differs from the final response, and may be adverse or advantageous. In spite of this complexity, there are general styles of response to stressors that are very well documented. In this section, the response of fluvial systems to stressors is characterized. This discussion is not comprehensive, but strives to demonstrate how fluvial systems respond to common stressors. Emphasis is placed on how the vital signs of fluvial systems may respond to stressors or how they may be monitored to better understand the effect of a stressor on a given watershed. With this background, appropriate methods can be selected to monitor fluvial vital signs depending on the needs and conditions the land area being managed.
Altered Hydrology
The hydrology of a stream system can change immediately in response to changes in land cover throughout the watershed. Anything that affects the volume or timing of water entering a stream will affect the flow regime and, subsequently, the channel geomorphology. Alteration of the hydrology of a basin may arise from situations such as changes in vegetation, which affects evapotranspiration rates; land development, which almost invariably causes a decrease in the infiltration capacity from the natural condition; or construction of storm water drains. Hundreds of studies have documented the hydrologic and geomorphic effects of development within a watershed. Four categories of general effects of development are now well known: alteration of hydrologic patterns, disturbance of stream geomorphology, degradation of water quality, and deterioration of stream habitat (U.S. Environmental Protection Agency, 2002; Cottingham et al., 2003). The specific response will vary depending on the degree of development (from a few roads and buildings to a fully urbanized watershed with storm drains) and the natural characteristics of the basin.
Changes in sediment transport and channel geomorphology will occur concurrently with changes in hydrology, or soon after. The spatial scale of the change will increase with time, starting at the area of highest impact. With respect to vital signs of fluvial systems, changewill most likely progress from changes in sediment transport rates, to bed configuration, to channel crosssection, and to channel planform and longitudinal profile (Knighton, 1998). This lag in reaction time to a stress is related to the energy expenditure necessary to cause the change (Ritter et al., 2002). In summarizing documented effects of watershed development on stream systems, Gregory and Walling (p. 295, 1987) state that “an increase in peak discharges due to the effects of urbanization could, for example, lead to an increase of channel cross-section size, an alteration of channel shape, and increase in the size of meanders and metamorphosis of planform along selected reaches from single thread to multithread as sedimentary bars accumulated.”
Altered Sediment Budget
Changes in the availability of sediment or the ability to transport sediment will immediately affect stream sediment transport rates and may affect all three channel vital signs: cross-section, planform, and longitudinal profile. The sediment budget may be altered simply by changes in hydrology—the driving force behind sediment erosion and transport. For example, development of a basin may affect the sediment budget in two general ways: (1) an increase in the amount of soil erosion and sediment input into channels due to an increased percent of overland flow in the basin, and (2) an increase in sediment derived from within the channel due to channel enlargement or extension caused by higher storm flow discharges. Alternatively, the sediment budget may be directly affected by natural or anthropogenic changes in land cover within the watershed that change the resistance or availability of soils and other surfi cial material (unconsolidated material or sediment on the land surface).
Consequently, a storm hydrograph (D) for an urbanized basin has a shorter lag time and higher peak discharge than a comparable forested watershed. Basin hydrology chart is adapted from Gregory and Walling (1987).
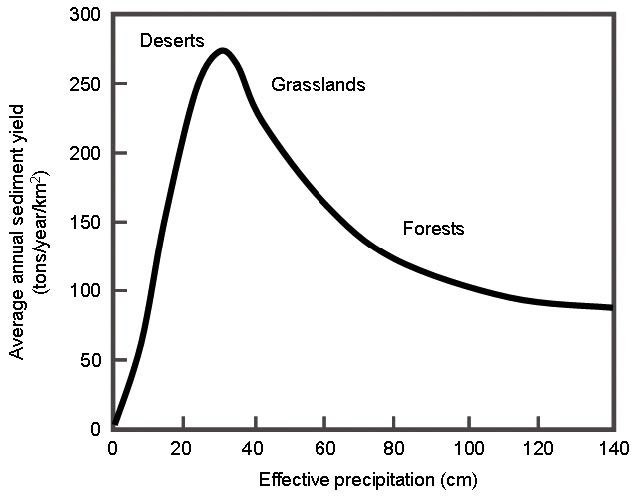
(Langbein and Schumm, 1958, Yield of sediment in relation to mean annual precipitation: American Geophysical Union Transactions, v. 39, no. 6, p. 1077. Copyright 1958 American Geophysical Union. Reproduced/modified by permission of AGU.)
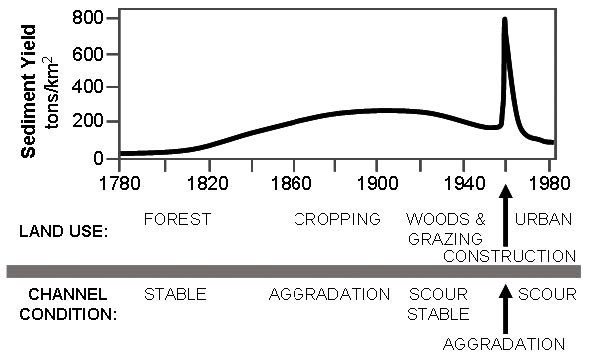
(modified from Wolman, 1967, A cycle of sedimentation and erosion in urban river channels: Geografiska Annaler, v. 49A. Reproduced with permission of Blackwell Publishing Ltd.).
Changes in the sediment budget caused by natural stressors, such as climatic change, landslides, or fires, clearly affect the sediment dynamics, but, in many cases, anthropogenic stressors can have an even more pronounced effect. For example, an estimated one-third of the sediment load naturally delivered to coastlines of the United States is trapped upstream of dams (Berger and Iams, 1996). Urbanization and general land development have been repeatedly shown to cause signifi cant increases in soil erosion and sediment transport, especially during periods of active construction. In a classic model of river adjustments to land development in the northeastern United States, Wolman (1967) related land use practices to sediment yield and channel condition (Fig. 9). He attributed a sharp increase in sediment yield and stream aggradation to a period of high construction rates. Numerous studies have corroborated the influence of construction on sediment yield. The sediment record in an impounded lake in the southern Appalachians showed a five- to ten-fold increase in sedimentation rates coincident with active home development in a small watershed (Miller et al., 2005). A change in land cover can also affect the sediment budget and channel form. For example, streams with a forested riparian zone in eastern Pennsylvania are wider and have slower lateral migration rates than nonforested riparian zones because of differences in the erodibility of channel banks (Allmendinger et al., 2005).
Changes in a watershed or stream sediment budget not only will affect sediment transport rates, but can affect water quality (e.g., turbidity), quality of streambed habitats, and channel crosssection (Cooke and Doorkamp, 1990; U.S. Environmental Protection Agency, 2002; Allan, 2004). As mentioned in the Basics section, channel pattern is controlled by the amounts and types of sediment load, and thus may change in response to shifts in the sediment budget of a stream. As with alterations of hydrology in a watershed, the system response time to a stressor that causes changes in sediment dynamics will vary, from immediate to 100 or more years, depending on the distance from the site of the stressor and the energy necessary for the reaction.
Direct alteration of stream channels largely results from anthropogenic activities, but can be caused by natural processes, such as landslides. Alteration of stream channels by humans dates back thousands of years. In Denmark, up to 98% of streams may have been directly altered from their natural condition (Brookes, 1995). This degree of alteration is much lower in the United States, especially in the generally rural areas of federally managed lands. Reasons for direct alterations of channels include flood control, straightening (channelization) to facilitate transportation or land development, and bank stabilization. Today, there is an increasing amount of direct channel alteration to restore and rehabilitate streams. Stream restoration attempts to return a channel to its natural, predisturbance condition. More commonly, streams are rehabilitated with the objective of partially restoring their structure and ecosystem function. The variety of direct channel alterations means that a simple summary of possible stream responses is beyond the scope of this chapter.
Nevertheless, in relation to altered channels and prior to the description of fluvial methods, it is instructive to recall that the natural channel form is a result of the sum of the conditions and processes affecting the stream and the watershed—that is, the climate, vegetation, topography, geology, and hydrology. Artificially altered channels designed without consideration of natural controls are highly unlikely to remain stable. In some cases, artificially straightened channels have regained a meandering pattern in ~100 years (Brookes, 1995) because of poor designs that do not account for the increased energy caused by the higher stream gradient and the tendency of many streams to meander. It is also worthy to note that many stream restoration projects have failed (Kondolf et al., 2003; Kochel et al., 2005). The high failure rates are likely perpetuated from simplistic approaches to classifying streams to predict stream behavior and select restoration approaches (Miller and Ritter, 1996; Kondolf et al., 2003; Kochel et al., 2005). In many cases, it requires significant expertise in fluvial geomorphology to assess the root causes of channel instability, determine the potential for restoration, and implement a successful restoration plan.
MONITORING FLUVIAL SYSTEMS
Overview
The six vital signs presented in this chapter are individual metrics for monitoring components of fluvial systems. All of the vital signs are interrelated, however, though certain ones may be more relevant in a given region than others. A monitoring program that is designed to assess changes in a single parameter may leave out investigation of a seemingly irrelevant component of the fluvial system. This section presents procedures according to three levels of intensity, which should enable land managers to customize a monitoring program by selecting their methods from a menu of options. A useful approach for designing a monitoring program is outlined by Reid and Dunne (1996). Their approach for rapid evaluation of sediment budgets is easily altered for use in a monitoring program for fluvial systems, and an example ofsuch an adaptation is presented in Table 2.
Three levels of methods or investigations for monitoring each of the six vital signs are described below. The general traits of these three levels are described in the introductory chapter of this book. For monitoring fluvial systems, as with other geologic systems, selection of a high-level method (i.e., level 3) does not negate the concurrent use of the lessintense, sophisticated methods (i.e., levels 1 and 2). In fact, in some cases, it would be impossible to carry out a level 3 investigation properly—either in design or interpretation—if a lower-level study had not been completed. After a brief description of how to design a study, this section provides a brief description of methods for each vital sign, then discusses how to approach a study to monitor the vital sign. Detailed descriptions of methods are abundant in books and journals (for example, Kondolf and Piégay, 2003), and, increasingly, through scientifi cally validated Web sites—for example, from the U.S. Geological Survey, the U.S. Environmental Protection Service, the National Park Service, and the U.S. Forest Service. We do not attempt to repeat this information here.
Design of a Study
In order to gather the information necessary to understand and properly manage a fluvial system, the study must be designed effectively. It may seem obvious, but an oft forgotten step in a study design is to identify the question that the monitoring is intended to answer. A poorly formed study question, without specific objectives, is likely to result in the collection of too little or too much data. In general, study questions in fluvial systems are rarely defined too narrowly. Alternatively, study designs are commonly too narrow because they fail to recognize the interconnectedness of fluvial systems.
A study design should answer the following questions.
1. What is the overall study question or purpose? (Is the question in response to a specific event, past action, proposed action, or problem where a targeted monitoring program is required? Or, is the purpose to establish a reference to identify or compare spatial or temporal trends?)
2. What are the smaller-scale questions that must be addressed to answer the larger question?
3. What information is needed to answer the questions? (Also, are qualitative or quantitative data necessary? What degree of accuracy is needed?)
4. What resources are available to address the question? (How important is this question compared to others that need to be addressed? How many people will be available? What is their skill level? How much time can the people commit? What equipment is necessary? How much money is needed? When does the study question need to be answered?)
5. What method should be used to gather the data?
6. Where should data be collected?
7. When and how often should the data be collected?
8. How will the data be analyzed and interpreted?
9. Can this study be piggybacked with complementary studies for improved effi ciency and understanding? (Water quality? Stream habitat? Hillslope processes? Soils?)
10. Will the results of the study be used as part of an adaptive management program? How?
11. Other? Additional questions pertinent to study design will arise due to the unique circumstances of the land or management—for example, safety, logistics, access.
Some of the practical matters that must be considered in any study design are summarized in Table 3 for all methods, including approximate cost, equipment needs, and skill level required.
METHODS FOR VITAL SIGN 1: WATERSHED LANDSCAPE
Synopsis
Changes in the watershed land cover will alter the hydrology and sediment budgets of fluvial systems and, therefore, the stream processes and form. The preliminary objective in monitoring this vital sign should be to identify regions of different land cover, especially those that are most likely to influence the path or rate of water flow through a watershed. If changes in land cover are observed or planned as part of management, predictions can be made about the potential impact on stream form and processes.
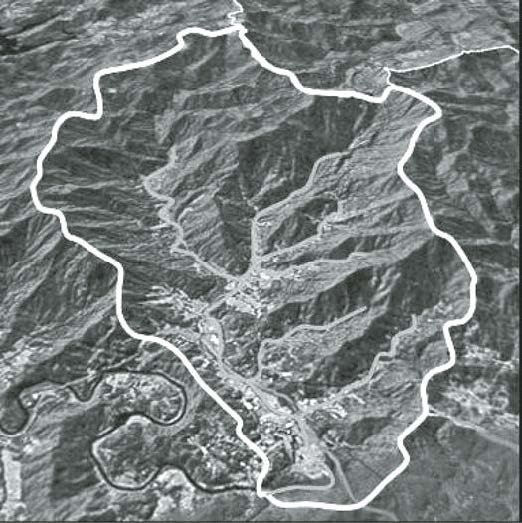
Methods
Level 1: Watershed Events Journal
Before any spatial analysis can occur, it will be necessary to define the boundaries of the watershed on a topographic map or scaled aerial image (Fig. 10). This process involves: (1) locating the mouth of the stream; (2) identifying all the topographic high points that separate that stream’s tributaries from its neighbors; and (3) drawing a line that connects these points. A detailed procedure for delineating a watershed by hand is described in detail
in Sanders (1998).
Land managers should keep a journal of all the activities that influence land cover, especially if spatial data are not available. The journal entry should include a description of the activity, whether the change is natural (fires, landslides, floods) or artificial (new construction, clear cutting), a description of its location, and the dates of the activity or event.
Level 2: Aerial Photographs
Level 2 investigations should include the calculation of drainage area and the surface area devoted to each different type of land use/cover. These calculations should be kept in a journal for the watershed of interest. This should be repeated any time there is a change in land cover or proposed change in land cover; otherwise this only needs to be done on a decadal scale.
The measurements can be estimated using paper maps and aerial photographs. A great deal of valuable fluvial geomorphic data can be stored for future analysis using carefully taken scaled photographs. Aerial photograph coverage is available for much of the country, and can be used reliably for larger rivers and watersheds to interpret channel planform, sinuosity, and major sediment sources such as landslides and forest fires. Aerial photographs must be taken at known elevations which can easily be converted into a photo scale. The simplest method for estimating areas from a map or aerial photograph with known scale is to: (1) highlight areas of interest, (2) superimpose a grid network, and (3) count the number of grids that fall within the highlighted boundary. Advanced methods to determine the area include using a planimeter or digitizing software.
Level 3: Geographic Information Systems
Geographic Information Systems are the tool used most frequently for spatial analysis, and should be incorporated in to an investigation of any level, if it is feasible to do so. A database should be constructed that contains information about all of the changes in land cover that can infl uence geomorphic processes that operate within the watershed. This would include information about bedrock geology, soils, slopes, hydrography, and vegetation type and density. This database can be used for identifying sensitive areas to study in greater depth, and to drive various types of visual and numerical models that relate precipitation to surficial processes such as runoff, groundwater flow, and soil erosion.
Study Design and Limitations (see Table 3)
Site Selection
Optimally, a watershed events journal (level 1), should be collected for all of the watersheds in the managed land. If this kind of coverage is not feasible, select watersheds for study in which either changes in land cover have occurred in the past, or are likely to occur in the future. For example, it would be important to collect this kind of data from watersheds that have been targeted for timber harvest or from those that have recently been affected by wildfires or landslides. In the case of watersheds that have already experienced a change in land cover, “before” and “after” data may be unavailable. In these cases, comparisons can still be made by substituting space for time. For example, to estimate the hydrologic impact of a new roadway on the sinuosity of streams downstream, reaches located upstream from the new parking lot can be used as a proxy for the (historical) “pre-disturbance” morphology and compared to reaches located downstream from the disturbance. Alternatively, comparisons could be made to neighboring watersheds with similar slope, substrate, aspect, and drainage area where similar disturbances have not occurred.
Scale Limitations
Land cover in large watersheds will obviously require more effort to measure than in smaller watersheds. The most useful approach to addressing the issue of watershed scale is to begin by quantifying land cover in low-order drainage basins and integrating these measurements to describe the larger watershed. Depending on the type and size of drainage network, data may be required for areas ranging from 100s of square meters for gully and rill systems in arid regions to 1000s of square kilometers for large fluvial systems. Obviously larger land areas require larger map and image coverage.
Time Limitations
In most cases it will be adequate to document changes in land cover using a series of “snap shots” taken at decadal intervals. However, in watersheds where catastrophic landscape changes such as landslides or forest fi res occur, it may be necessary to reassess the land cover immediately following the disturbance event and then annually as vegetation cover is reestablished and new sedimentary deposits or soils begin to form. A similar approach should be taken when studying the effects of timber harvesting or the construction of new buildings, parking lots, and roads.
Because most spatial analysis involves map, air photo, and/or satellite image interpretation, investigations that are based on these approaches do not typically have many time restraints. However, if a project requires the construction of maps or the acquisition of new photographs, some thought should be given to the timing of these projects. For example, a large-scale series of aerial photographs that are to be used to measure the surface area of roadways should not be scheduled for a time of year when foliage from trees growing along the roadside will obscure the road. Autumn may not be useful either since fallen leaves or snow may obscure the road. Late winter or early spring may be the most optimal time to collect these data to minimize the influence of these factors.
Synopsis
The basic purpose for monitoring watershed hydrology is to document relationships between precipitation, groundwater, and stream flow. As discussed in the introductory section, any change within the watershed, natural or artificial, is likely to have an immediate effect on the hydrology. Hydrologic data can be used to identify flood-prone locations in the watershed, to estimate likelihood of channel forming events, and relate data to sediment transport.
Hydrologic data are commonly presented in a hydrograph, which relates stream discharge to time. Frequently, hydrographs for wells or streams are compared with precipitation intensity to produce storm hydrographs. These graphs are used to demonstrate a river response to rainfall events, and provide useful data for understanding the interaction between a stream network and the atmosphere, and the stream channel and its floodplain (Fig. 7D).
Discharge for a stream is traditionally calculated using a simple continuity equation based upon hydraulic geometry:
Q is the stream discharge (L3T–1)
A is the cross-sectional area of the channel that is occupied by water (L2)
V is the water velocity (LT–1)
This equation can be replaced with a fl ow regime equation that is based simply on the width of the channel:
Q = aWb
Q is the stream discharge (L3T–1)
W is the width of the channel (L)
a and b are unnamed constants
The use of this equation requires calibration for different watersheds since they rely on unspecified values of two constants. The constant a contains variables that may vary from one location to the next within a single watershed (Parker, 1979) and can be expected to vary on the watershed and regional scales as well. Because of its importance, discharge is related to many stream characteristics and can be used to understand stream stability (see summary in beginning of this chapter).
Methods
Level 1: Hydrologic Events Log
Thousands of stream and precipitation gauges are established across the United States and likely exist in or nearby most managed land areas—many of these gauges have decades of records. If present, level 1 investigations begin with compiling a record of rain and discharge data. The U.S. Geological Survey stream gauge data are accessible through its Web site; many sites include statistics on average and flood flow discharges. Using these data, a database for hydrology can be assembled to calculate statistics for both storm and flood frequency in the area of interest. They can also be examined for patterns that may be related to climatic change or land use change.
In addition to these data, it is important that simple observations be made with respect to the date, location, extent, and duration of all overbank (flood) events in the areas of interest.
Level 2: Hydrologic Monitoring
More complex studies will require further instrumentation than that collected with level 1. Staff gauges should be installed throughout in the direct area of interest. (A staff gauge is simply a relative water-level indicator established on a permanent feature such as a bridge, tree, or post.) This will permit the collection of quantitative data, which though not as valuable as stream discharge data, can be used to identify patterns of hydrologic response. In addition, precipitation gauges should be installed at multiple locations throughout the watershed; large, complex watersheds will require more gauges than small, simple watersheds. These gauges should be monitored routinely, but especially during and after precipitation events. The frequency of monitoring will depend on the study design and question.
When overbank flows occur, maps should be constructed of the maximum extent of the water surface using flood debris or other such indicators. After enough hydrologic data has been collected along the stream of interest, standard methods can be used for flood frequency analysis (Bedient and Huber, 1988).
Level 3: Hydrologic Stations and Data Analysis
In addition to level 1 and 2 data, a network of more sophisticated gauges should be installed for continuous monitoring of precipitation, river stage, and water table elevation. More detailed studies of fl oodplain hydrology could include a variety of data collected with specialized tools. For example, tipping bucket rain gauges can be fitted with data loggers to monitor precipitation intensity during rainfall events. Repeated measurement of water levels in nested piezometers could be used to monitor changes in the elevation of the water table, to demonstrate how hydrology changes with depth, and to demonstrate rates of flow toward or away from the channel. Defining the nature of the interaction of groundwater and stream water is very important to stream ecosystems.
In order to calculate stream discharge from river stage data, it will be necessary to measure water velocity and survey cross-sections where water-level recorders are located. Standard U.S. Geological Survey stream gauging stations record stage in 15-minute intervals. The stream stage data can be related to stream discharge by the establishment of rating curve—an empirical curve that relates discharge to stage by repeated measurements of actual discharges at a variety of flow conditions. Establishment of a rating curve requires the measure of velocity and depth at numerous points in a stream (Fig. 11). Velocity measurements are commonly made with specially designed instruments such as the pygmy or AA meter. These devices are held below the water surface at 6/10 of the river depth, where flowing water causes a propeller to spin. Electromagnetic flow meters and acoustic Doppler velocimeters can also be used to determine velocity (Kondolf and Piégay, 2003).
Study Design and Limitations (see Table 3)
Site Selection
Since precipitation can vary signifi cantly with microclimate, simple geography (rain-shadow effects, etc.) as well as specific geomorphic characteristics such as elevation, slope, and watershed aspects can have a dramatic infl uence on the volume of precipitation accumulating at any single location. Controlling these variables during the installation of rain gauges can greatly reduce the amount of error associated with the study results. Groundwater wells should be installed along a line perpendicular to topographic contours. Optimally, the wells should be deep enough to sample water below the water table during
late summer and fall. A line of groundwater wells should begin on colluvial slopes (hillslopes mantled by material transported and deposited primarily by gravity) in the recharge zone of the watershed where soils are thin, and continue across the alluvial floodplain.
Stream hydrology should be monitored in stable reaches of streams that are free from obstructions such as boulders and large woody debris. It is also wise to minimize the effect of bedform roughness by limiting studies to cross-sections with uniform depth, such as in large riffles or chutes. In streams where the channel may be too shallow to measure velocity during low flows, select cross-sections to monitor where the flow is constricted. For example, if a large boulder that signifi cantly decreases the effective width is present along one bank, depth will be slightly higher in that location. Regardless of where stage is measured, it will be necessary to survey the cross-section to make discharge calculations.
Scale Limitations
Investigations of precipitation and groundwater flow in large watersheds require a large number of instrument stations. Floods in higher-order streams are caused by seasonal snowmelt or long duration, low-intensity storms that influence large areas of Earth’s surface. This means that a large network of rain gauges is required to acquire useful rainfall data for large watersheds. Since the elevation of the water table is determined by local rainfall, similar reasoning can be applied to studies of groundwater flow in large watersheds. During intense precipitation events, the rainfall from a single storm may only fall over a single small watershed, causing short, high-intensity floods. Monitoring rainfall and groundwater flow in a small watershed requires fewer instruments, but since small watersheds are more abundant than large ones, the scale of the study is determined by the number of small watersheds to be monitored.
There are very few low-order watersheds with stream gauges in them, despite the fact that most sediment produced in a watershed comes from these streams. Budget limitations frequently demand the use of a small number of gauges, making it less feasible to monitor stream hydrology in small watersheds. Waterlevel recorders work just as well in large streams as they do in small ones, but it is more challenging to survey cross-sections in large rivers.
Short-term studies of hydrology rarely provide realistic records for a watershed since variance over the short term is subject to minor fluctuations in weather events. Longer records are required to determine statistically significant return intervals for precipitation and flood events. Where long-term records are not available, it is frequently possible to establish a relationship between data from a gauge with a short record to data from a nearby gauge with a longer record. Using that relationship and the longer record, historical data for the short-record gauge may be reconstructed. New hydrologic data should be collected on a daily basis throughout the year, but this data collection from gauges or loggers takes very little time.
Cost Limitations
Level 1 investigations require only data that are readily available online. Monitoring at this level can be done at no cost if a computer is available with Internet service. Level 2 investigations require the use of basic tools such as simple rain gauges and staff gauges. This equipment is inexpensive ($10 to $100; all amounts herein are in US$) and easy to acquire or construct using materials found in any hardware store. Level 3 investigations require more sophisticated instruments including tipping-bucket rain gauges ($100 to $1,000), stilling wells with water-level recorders ($1,000 to $10,000), groundwater monitoring wells ($100 to $1,000), and data loggers ($1,000 to $10,000).
METHODS FOR VITAL SIGN 3: SEDIMENT TRANSPORT
Synopsis
Sediment contamination is the largest source of pollution in our country’s waterways. Sediment itself may be the problem when it is deposited in some location in too much abundance, or when it resides in the water column, blocking out light from aqueous ecosystems. As discussed previously, changes in land cover will likely change the sediment budget of a stream system, which, in turn, may cause a change in channel form or type.
Sediment transport data are normally presented on a rating curve, which shows how sediment concentration changes over time. Since there is so much temporal variance in the transport of sediment, there is little value in measuring sediment without knowledge of the date, time, and hydrological conditions when the sample was taken. Further, a single measurement or a few sporadic measurements are not particularly useful either, since sampling from a variety of discharges is required to construct credible rating curves. Measurement of sediment sampling, unless automated, is time intensive; because of this, mapping of bedload material can be used to make indirect inferences about sediment transport (see Vital Sign methods for Channel Planform and Longitudinal Profile).
Methods
Level 1: Sediment Transport Event Logs
A log of observations of characteristics of sediment transport can be made during rain or snowmelt events in a manner similar to that described for the hydrology vital sign. The observations needed depend on the type of sediment load (suspended or bed) important to the study problem. Qualitative observations of turbidity can be made by simple comparison of stream water turbidity to standards. Visual observations can be made of sand and gravel movement in smaller streams where the bed remains visible. To be of any value, these observations must be linked to stage or discharge data.
If the channel bed cannot be observed during high flow, then observations of sediment sizes and distribution on the channel bed at areas of interest can be made after the event.
Level 2: Stream Sediment Load Sampling
Any quantitative assessment of sediment transport will require direct sampling and analysis of stream water under a variety of conditions. Because high flows are of the most geomorphic significance, safety is a common concern during sampling.
Bedload samples are commonly collected with specially constructed nets or traps. The sampler is placed on the bed of the stream for a specified time interval, and then removed. The most commonly used instrument used for discrete samples is the Helley-Smith sampler, which is little more than a sturdy net with a metal screen (Fig. 12B). The sediment is emptied into a container and transported to the laboratory where it is sieved and weighed. Suspended sediment samples are collected in a similar way. A depth-integrated sampler consists of a torpedo-shaped metal housing, a wading rod, and a plastic sample bottle. The DH-48/59 depth-integrated sampler is the standard tool for this job (Fig. 12C). The operator starts at the water surface and slowly lowers the sampler to the bed and then back to the top at a constant rate, recording the length of time the bottle has been submerged.
The sample bottles are then transported to the laboratory, where the solids are filtered out and weighed.
Level 3: Automated Stream Load Sampling and Analysis
Various high-tech tools are available to monitor sediment flux and its influence on stream hydrology on a continuous basis. This information can be used to determine sedimentological responses to rapidly changing hydrologic conditions associated with intense storms, or releases of water from reservoirs upstream. When used with hydrologic data from gauging stations, these instruments provide scientists with extremely accurate sediment rating curves. Multiparameter recording instruments can be placed in strategic
locations to collect measurements of turbidity, total suspended solids, and total dissolved solids on a continuous basis. Similar data can be collected for bedload transport through the use of bedload traps, which are placed on the streambed and emptied periodically while hydrologic conditions are being measured. The usefulness of data from bedload traps is somewhat limited since the time resolution of the record is limited by the frequency with which the traps are emptied. There are a few places in the world where conveyor-belt–driven bedload sampling traps are in use, but the expense of these tools makes them impractical for most monitoring projects.
Study Design and Limitations (see Table 3)
Site Selection
Sediment transport investigations should be conducted in sampling areas with relatively homogenous bathymetry (topography of the streambed), and without major obstructions such as boulders or large woody debris. Sampling reaches should have low sinuosity and the banks should be free of vegetation that might alter the flow velocity. If bedload sediment is the focus of the study, then the study reach of the stream should be divided into like sections with similar observations made in each section.
Scale Limitations
Sampling sediment in large rivers may require specialized equipment that must be permanently installed on channel banks. Representative locations across the width of the channel are selected, and sediment concentration or volume from discrete samples in several theoretical stream tubes is averaged to determine the total sediment discharge. This approach, while similar to that used for smaller streams, will require much more effort and time in large ones.
In order to construct a useful sediment rating curve for a stream network, multiple samples must be taken from multiple locations on a daily basis. Since a great deal of laboratory work is required for grain-size analysis of a large number of samples, it may be impractical to do more than measure sediment concentration. Likewise, the usefulness of data from bedload traps will depend on the frequency with which the traps are emptied. Bedload samples are extremely difficult to transport to a laboratory, so daily analysis in the field may be required. Remotely sensed data using acoustic samplers, on the other hand, may only require a few infrequent visits to the field site to download data.
METHODS FOR VITAL SIGN 4: CHANNEL CROSS-SECTIONAL GEOMETRY
Synopsis
Repeated surveys of channel cross-sections can be used to quantify channel enlargement, channel migration, and the volume of sediment deposited or eroded from the bed and banks. Channel cross-sections can be compared with others from different locations at a single time to assess physical characteristics of different reaches, or from different times at a single location to monitor channel change. Changes in cross-sectional area measured at multiple locations can be integrated over the length of a study reach to construct a sediment budget for a channel network as part of a long-term investigation of stream response to changes in watershed land cover or sediment yield.
Methods
Level 1: Repeat Photography and Observations Log
Simple investigations of stream cross-sections begin with descriptions of bank characteristics, commonly with use of a template for characterization and/or classification, at the sites of interest. Characteristics that should be recorded include channel shape, evidence of erosion or deposition, and bank geometry. In addition, scaled photographs should be taken from several vantage points, including views of the cross-section taken from upstream and downstream with the photographer standing in the channel. Locations should be plotted on a map and/or aerial photograph, and located with a global positioning systems (GPS) unit.
Level 2: Cross-section Measurements of Key Features
Measurements of channel width, depth, and key geomorphic features add to the quality of data of level 1. Width should be measured relevant to a geomorphically stable reference point or datum, but should be made at as many different points as are present (Fig. 13). Widths should be measured by stretching the tape perpendicular to the average flow direction. The simplest and most effective depth measurement is the maximum depth along the cross-section between the elevation of the selected datum and the bed of the stream. These data can be augmented by careful repeat photography so that changes in channel cross-section can be quantified.
Level 3: Repeat Surveys at Monumented Sites
Repeated surveys of monumented cross-sections are the most effective approach to studying change in channel dimensions. The end points of a cross-section can be monumented with a ½-inch steel rebar pin driven into the ground at each end of the channel cross-section. Their positions should be mapped with precise distances and bearings to landmarks, such as large trees or boulders, so that they can be found easily during future visits. Channel cross-section data can be collected with survey equipment or with a tape measure and survey rod. Enough data points should be collected to fully define the geometry of the channel and show key geomorphic features (e.g., channel bank, slumps, sediment bars). If detailed aerial photographs are available, channel dimensions (width) can be measured at many places rapidly. These data will be most valuable if historic aerial photographs are available, so that rates of change can be determined.
Study Design and Limitations (see Table 3)
Site Selection
Multiple cross-section sample sites will need to be established, whether the study purpose is for targeted or trend monitoring, because of the high variability in channel cross-sections. At least one cross-section site should be established for each similar reach of the study stream. Study sites for targeted monitoring of channel geometry should be in locations where recent and persistent change is evident, as these are likely of highest management concern. Figure 14 depicts characteristics of a dynamic crosssection stream section with evidence of scour on the cut banks, a general deepening of the channel toward the scoured bank, a pronounced ridge atop the inner bank deposit, and a pronounced depression between the ridge and the valley flat adjacent to the inner bank.
If the goal of a monitoring project is not to document changes in channel form of a single stream, but to document differences between multiple streams, study sites may be located in more stable reaches and compared using the ergodic approach (see section on watershed land cover). Cross-sections in a nearby watershed with similar drainage area, slope, bedrock geology, substrate, and aspect can be compared with respect to average dimensions such as width, depth, the width:depth ratio, entrenchment ratios, and cross-sectional area.
Scale Limitations
The type of equipment required for monitoring cross-section geometry depends to a large extent on the width of the channel. Channels as wide as 20 m may be measured effectively using simple measuring tapes and an auto level. For larger channels, the length of the section may make it difficult to keep the tape straight and level. Some of this sagging may be addressed by switching to a steel tape or tag line. Larger channels may also require the use of more sophisticated survey equipment. Cross-sections in large rivers are measured from boats using sonar devices to record depth soundings.
Time Limitations
As with measurements of the longitudinal profile, the temporal resolution of cross-section measurements depends to a large extent on local hydrology and substrate type. For most alluvial streams, an annual or semi-annual resurvey should be adequate to monitor channel position and geometry. Cross-sections of bedrock streams will likely change minimally on an annual basis. Similarly, if low flow dominates for a number of years, crosssectional geometry of alluvial streams may not change much at all. Straight reaches of alluvial streams can be remarkably stable over long periods of time, making it unnecessary to repeat surveys frequently. On the other hand, a very flashy stream (characterizedby rapid changes between peak and low flows) may scour its banks with great frequency, requiring repeat surveys to be conducted after any high flow event.
A single person can compile descriptions and maps of bank morphology using U.S. Geological Survey topographic maps ($10 to $100), a customized classifi cation scheme, and a digital camera ($100 to $1,000). It may require two people to measure channel dimensions with a measuring tape ($10 to $100) or a laser range finder ($100 to $1,000). Surveys of channel cross-sections can be measured using an auto level ($1,000 to $10,000) for narrow channels, or a total station or survey-grade GPS ($10,000 to $100,000) for broader channels.
METHODS FOR VITAL SIGN 5: CHANNEL PLANFORM
Synopsis
Stream channel planform can vary signifi cantly in response to changes in parameters such as stream gradient, discharge, or sediment load. Through careful analysis of channel planform it is possible to identify reaches where erosion, deposition, or transport processes are dominant. Analysis of channel planform provides data needed for determining morphological characteristics such as pool spacing and meander intensity. Channel planform data are usually presented in the form of maps or aerial photographs. Maps or photos representing multiple time steps are compared to determine changes in sinuosity and overall channel form.
Detailed maps can be used for a variety of purposes, but are most commonly needed to document important spatial relationships in the riparian zone, or to illustrate dramatic shifts in channel position, planform, or sinuosity. For example, maps of riparian vegetation types may reveal information about soils and hydrology; maps of sedimentary deposits in the riparian zone could be used to demonstrate the extent of flood water; and maps of puddles and puddle depressions can reveal information about flood hydrology. A series of maps of features such as terraces, avulsion channels, levees, bank tops, channel bars, or water surface limits can be used to document rates of bank erosion, changes in channel curvature, or the transition between a single thread and a braided channel.
The type of map required depends upon the scale of interest and the properties to be mapped. Simple two-dimensional maps can be constructed using a variety of different techniques that require varying levels of expertise. Pace-and-compass can involve a significant amount of error from imprecise bearing measurements and pace-length estimates, even under ideal conditions. Two-dimensional maps are sufficient for demonstrating spatial relationships in the channel and riparian zone, but for more detailed geomorphic maps, a three-dimensional method may be required. For example, three-dimensional maps can be used to measure the volume of sediment in channel bars or the height or depth of important features such as fl oodplain levees or stream banks.
Methods
The planform type can be determined using maps of the channel or aerial photography. The channel can be classified in terms of the number of channels, bed topography, presence, type, and abundance of bars, and the sinuosity. When describing channel planform, the field person should classify the channel planform type as either single thread, sinuous or straight, single thread meandering, braided, or anastomosing. Floodplain and channel characteristics can also be documented using scaled photographs of each of these elements. Initial field surveys can be repeated in subsequent years to document any changes in conditions. Field observations of selected sites should include locations of sediment bars, pools, and knickpoints.
Channel sinuosity is easily measured using U.S. Geological Survey topographic maps for long reaches of a river, and largescale aerial photographs for shorter reaches. Sinuosity is simply a measure of the ratio between the length of the channel and the straight-line distance between the same two points (Fig. 14).
Level 2: Historical Analysis
At this level, more detailed comparisons can be made using historical maps and air photos, with particular attention paid to changes in overall pattern, rates of lateral migration, and sinuosity. Using simple tools such as those mentioned in the level 2 investigation of watershed land cover, the size and number of channel bars can be measured and compared from one year to the next. Also, shifts in channel position can be noted and measured. If aerial photographs are available for different years during the same season, changes in vegetation on the floodplain and on channel bars can be also be noted. Repeated field observations should note changes in bed topography and features, and permit the determination of pool sizes and spacing.
Sketch maps should display the position of the channel(s), the width of the floodplain, the type and dispersion of vegetation, the size and location of different types of sediment deposits, channel obstructions such as large woody debris or bed structure, the location of any standing water, muddy depressions, or avulsion channels. Vegetation maps should include the location of large trees and clusters of shrubs, and the distribution of herbaceous vegetation. Maps of large sedimentary features should denote the shape, size, and strike of the deposits, and the map symbol should designate whether the sediment is predominantly gravel, sand, or mud. Maps can be completed on enlarged aerial photographs or topographic maps, or by pace-and-compass maps.
Level 3: Image Analysis and Mapping
Advanced spatial analysis of the channel planform can be conducted using remotely sensed data acquired from low-altitude reconnaissance flights, or from satellite images. Annual surveys of extended reaches can be conducted to measure channel geometry in detail, to evaluate changes in bank form, and to determine rates of channel migration. Detailed topographic maps of the channel bed will provide more precise measurements of pool and riffle spacing, size, and form. They can record the positions of bank tops, channel bars, vegetation boundaries, sediment deposits, and water surface edges. Dating of vegetation, or dendrochronology, on sediment bars can be used to establish a time frame for origin of key features. Planform maps can also be used to estimate the surface area of channel bars, the water surface, or the riparian zone. Collection of these data would likely be done in tandem with monitoring methods for other vital signs (e.g., longitudinal profile).
Study Design and Limitations (see Table 3)
Site Selection
In order to monitor pertinent changes in channel form, it may be necessary to collect data from any reach in the stream network that isn’t confined by bedrock. Initial analysis using maps and photos will help to identify reaches where change is most likely. These reaches should be mapped in greater detail, or with a greater frequency. For most streams, analysis of planform characteristics need only be done at several-year intervals or after key hydrologic or sedimentation events. Bedrock systems will require less frequent monitoring.
Scale Limitations
Low-order streams in forested watersheds are poorly suited for analysis using remote sensing data. They are, however, much easier to survey and map in the field than larger streams. While larger rivers are more difficult to map in the field, they are easier to study using aerial photography and satellite images. The size of the streams being investigated will determine the most appropriate form of remote sensing data. The resolution of satellite images is good enough for investigations if useful data has been acquired for a large river (more than 200 m wide), while aerial photographs are more useful for smaller streams (Gilvear and Bryant, 2003).
Cost Limitations
Air photos are often easy to acquire, but data from a useful coverage over sequential years may be cost prohibitive ($1,000 to $10,000). Digital versions of the same coverage may be available at a lower cost on CD ($10 to $100), but this may require an investment in software ($1,000 to $10,000) that provides the analyst with tools for interpreting the images. Having a large-scale set of aerial photographs taken of a watershed is an option for regions
where coverage does not already exist. The cost of acquiring these images is a function of the size of the area to be photographed and the desired scale of the photos ($1,000 and up).
METHODS FOR VITAL SIGN 6: CHANNEL LONGITUDINAL PROFILE
Synopsis
A longitudinal profi le is an x-y plot showing bed elevation as a function of downstream distance. Surveys of the longitudinal profile of a stream are used to identify locations where (1) sediment is being produced from bedrock exposed in streambeds, (2) bed sediment is accumulating, and (3) channel incision is occurring. By resurveying these profiles, it becomes possible to estimate volumetric changes in the transfer of bed sediment from one reach to another and the production of new sediment from bedrock erosion. Longitudinal profi les can also be used to monitor changes in pool spacing, the progress of a sediment pulse from a landslide through the watershed, or upstream migration of knickpoints.
The upstream migration of knickpoints in streambeds has been related to rates of denudation (landscape lowering). In tectonically active regions of the country, rates of knickpoint migration may be related to rates of tectonic uplift. Bed aggradation and degradation typically refl ects changes in sediment input from upstream. Development or deforestation, for example, may cause changes in hydrology that could result in increased rates of deposition and erosion. Similarly, a channel with an increasing abundance of bedrock exposure in the bed may indicate elevated rates of bed scour or channel incision.
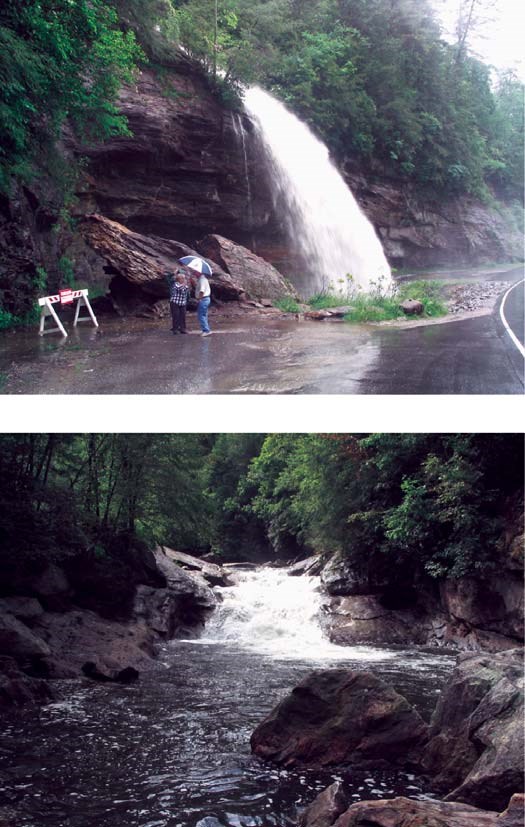
(Bottom) Another knickpoint just downstream from the first picture in this figure. This is an unscaled photograph of a scene that is frequently photographed by tourists from the region, making it useful for comparison with scaled photographs.
Level 1: Profile Characterization
The locations of bed features such as knickpoints, bedrock, and major pools and bars can be documented by marking on aerial photographs and/or maps, or using photographs and/or maps, or using a mapping or recreational grade GPS. Large knickpoints in streambeds (such as the waterfalls shown in Fig. 15) are frequently photographed by park visitors, especially when they are located near roadways and popular trails. Scaled photographs can be taken at these locations to graphically demonstrate the height of knickpoints, the spacing of pools, or the character of bed materials. The approximate location of non-bedrock knickpoints is most important because it is generally an indicator of channel instability.
Level 2: Profile Mapping
Simple mapping of selected stream reaches can be done with tools such as hand-levels, tape measures, and range finders to document the bed slope, riffles, pools, steps, bed materials, and knickpoints. When constructing simple sketch maps of a segment of a stream, field technicians should use standardized map symbols to characterize bed sediment material, pool depth, and the direction and type of stream flow (Fig. 16). Additionally, it may be desirable to include information about the riparian zone on these maps including floodplain vegetation, standing water, or landmarks such as large trees, channel bars, or large woody debris.
(D) In riffle-pool streams, flow is turbulent in riffles, and becomes more laminar over pools. Laminar flow conditions are locations where finer sediment is commonly desposited due to weaker cross-channel and vertical currents.
More precise measurements for bed features identified in level 2 can be collected using more sophisticated tools. At this level, a mid-channel line is surveyed at regularly spaced intervals over the entire length of the channel using an auto-level, a stadia rod, and a measuring tape. The appropriate approach for surveying a longitudinal profile depends on the specific goals of the monitoring project. For example, surveying only a few geomorphically significant points along the profile can suitably depict the height of knickpoints, or the pool spacing in a small reach of the stream (Fig. 17) if the goal is simply to determine the average dimensions of these features, while a survey of the entire channel may be required to identify regions of the stream where pools are anomalously shallow or absent (Fig. 18).
Surveying the upstream ends of riffles (2) should illustrate pool spacing, and including the deepest part of the pools will illustrate the bed relief.
Study Design and Limitations (see Table 3)
Site Selection
If the study goal is for targeted monitoring of an unstable reach, data on the longitudinal profile can be invaluable at monitoring changes because slope directly relates to hydraulic energy—all else being equal, steep slopes have more energy and are the most likely location of change. If the study area is too large to be mapped, then representative reaches should be selected for study. Longitudinal profiles provide measurements that are also necessary for making predictions about sediment transport and hydrology. Future changes in land cover will result in some degree of change in profile, so a baseline survey will be a valuable resource. Longitudinal profi les should be collected in any watershed where dramatic change is anticipated, or where it has occurred in the recent past. For example, these might include watersheds with anomalously high sediment yield, numerous sediment bars, abundance of bedrock exposures, or a large number of knickpoints.
Scale Limitations
High-order streams may be too large to survey using the methods described above. Large rivers may require the use of boats with sonar equipment to retrieve depth soundings. Longitudinal profiles take serious effort, even in smaller rivers. For this reason, it will probably be most effective to construct the profile using widely spaced points at first, and then survey with greater detail in small reaches with knickpoints, or unusual pool sizes or spacing.
Time Limitations
In the case of most undisturbed “natural” streams, changes in longitudinal profile may only be noticed over long periods of time. For these streams, it may only be necessary to repeat these measurements once every ten years. If the stream has indicators of instability or has experienced a disturbance such as a landslide, dramatic change in land cover, or intense precipitation, it may be necessary to repeat measurements on an annual basis, or even more frequently. For example, alluvial knickpoints can migrate rapidly upstream, especially in arid or semi-arid regions where meters of migration can occur in a single event. Alternatively, riffles and pools are able to migrate downstream or expand and contract over time, depending on the intensity of the discharges they are subjected to. A sediment pulse deposited by a landslide may move downstream through translation, dissipation, or a combination of both. The appropriate sampling interval for studying these kinds of processes will vary, and can only be determined through frequent visits to the reaches of interest.
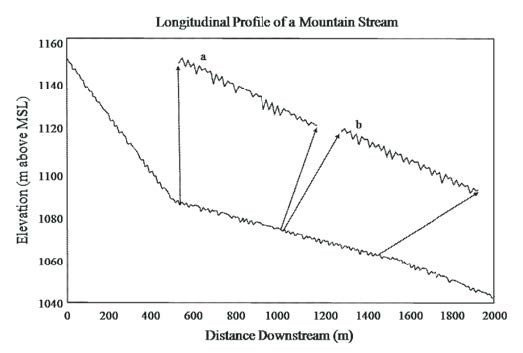
Stream-walk mapping of knickpoints and bedrock outcrops can be conducted by a single person using U.S. Geological Survey topographic maps, a Jacob staff, and a laser range finder. The cost associated with this type of investigation is therefore relatively low ($100 to $1,000). More precise maps can be constructed with optical surveying devices such as automatic levels or mapping-grade GPS ($1,000 to $10,000). More advanced survey equipment such as traditional or robotic total-stations ($10,000 to $100,000) require a clear line of sight. Survey-grade GPS ($10,000 to $100,000) do not require a clear line of sight, and are almost as accurate as total stations.
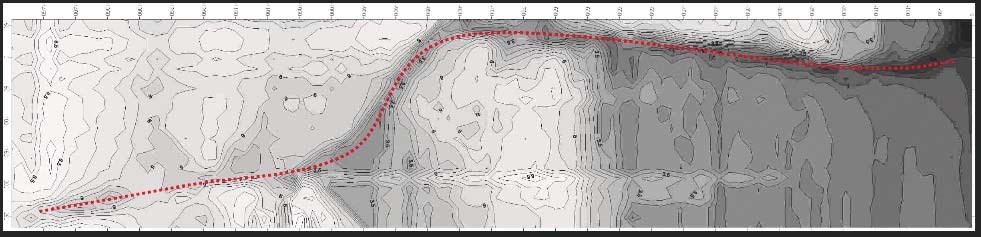
Overview
A case study is used here to demonstrate some of the methods and approaches reviewed in this chapter. The project described here has likely involved a larger budget and more personnel than most land managers will have available, but, nonetheless, does exemplify many of the principles, methods, time-scales, system characteristics, definition of study goals, assessment of needs, choosing of methods, and interdisciplinary cooperation that should ideally be used in many studies. The study described here is ongoing, but much of the work has been published in a book, Great Basin Riparian Ecosystems: Ecology, Management, and Restoration (Chambers and Miller, 2004).
Riparian corridor and meadow ecosystems in upland watersheds are of local and regional importance in the Great Basin. Covering only 1–3% of the total land area, these ecosystems contain a disproportionately large percentage of the region’s biodiversity. Stream incision is threatening these sensitive ecosystems. The downcutting by streams lowers the groundwater table and results in a vegetational shift from wet meadow species to drier communities. Many of these upland watersheds are located in the Humboldt-Toiyabe National Forest. Understanding the structure and function of riparian meadows and the impact of stream incision on these ecosystems has been a concern of the USDA Forest Service. A multidisciplinary research team of scientists from government (U.S. Forest Service and U.S. Environmental Protection Agency) and academia has been working to better understand the geologic and geomorphic settings, surface and subsurface hydrologic regimes, vegetation patterns, and interconnections of biological and physical systems of upland riparian meadow systems in central Nevada. This information is also being used for prioritizing ecosystem degradation and restoration potential to better direct available resources and evaluate the success of restoration alternatives. Brief descriptions of some the study questions, methods, and results are described below.
Methods. The geologic, geomorphic, and hydrologic setting of upland riparian meadows was evaluated throughout five mountain ranges of central Nevada to learn the conditions necessary to support riparian meadows. These methods included mapping in the field and on aerial photographs and installing and monitoring groundwater wells. The stratigraphy of modern and ancient stream sediments was studied in conjunction with the geomorphology to establish a long term record. The longitudinal profiles of streams were characterized using level 1 and 2 methods (profile characterization and mapping, repeat photography).
The channel has widened since then (2004), but the channel bed elevation appears to be stabilized because of a coarse-grained channel bed. Photograph by Jeanne Chambers.
Repeat photography of active knickpoints demonstrates rapid changes in the channel and an unstable system (Fig. 21).
Methods. Simple (level 1) field mapping techniques were used for many streams in basins supporting riparian meadows. These methods included determination of stream cross- sections, longitudinal profiles, bed materials, and degree of incision (Fig. 22). In addition, the geologic and drainage basin morphometric characteristics of each study site were determined.
Results. Based on the field data, stream systems were categorized into different sensitivity groups—that is, some basins are much more likely to undergo incision if the systems are stressed by natural or anthropogenic causes. The variation in the nature of system response appears to be controlled by geology, drainage basin morphometry, and watershed hydrology (Germanoski and Miller, 2004). This information is valuable to help prioritize management and restoration activities. Sequential aerial photographs show rapid upstream migration of channel systems into a previously unincised wet meadow complex (Fig. 23).
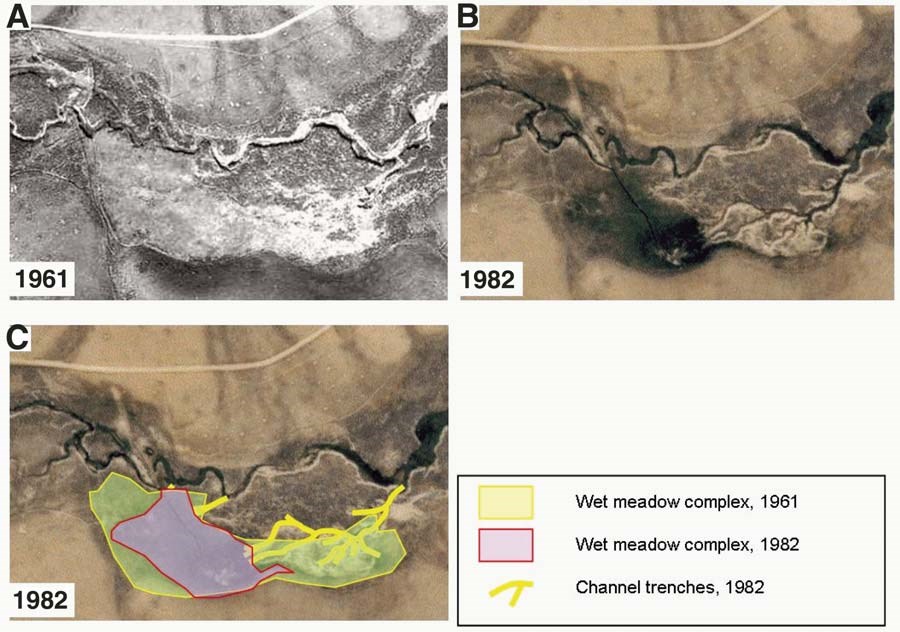
Note absence of any channels in main part of meadow in 1961 (A) and meadow complex loss by 1982 (B) due to incision of meadow by headcut migration of channel trenches (C). Example headcut is shown in Figure 24C.
Methods. Mapping and classification of vegetation, installation and monitoring of groundwater wells, and stream discharge measurements were used to understand the hydrologic characteristics of sites, groundwater-stream water interactions, and relationships to vegetation.
Results. A strong correlation exists between types of meadow vegetation and the depth of the groundwater table below the ground (Fig. 24) and some stream characteristics (e.g., depth of incision, bed material grain size). Stream incision is immediately followed by a drop in the groundwater table and, with some lag time, a change in vegetation type (Chambers et al., 2004; Jewett et al., 2004). Understanding the relationship of groundwater, stream water, geomorphic setting, and vegetation can be used to: (1) assess the impacts of existing stressors, (2) make predictions about potential impacts from stressors, (3) select best management or restoration options, and (4) estimate the likelihood of success of rehabilitation options.
Monitoring fluvial systems can be a daunting task because of the scale and interconnectedness of river systems. The form and processes of a stream are a response to most natural and artificial processes and properties within the watershed. They are also subject to external factors—most notably climate. Recognition of the importance of streams continues to grow as the relationship to ecosystems and land use activities increases. There are dozens upon dozens of methods to monitor stream systems. A monitoring program, more than an understanding of the methods, requires an understanding of the basics of fluvial geomorphology, characteristics of systems, the types of stressors that are most likely to drive change in fluvial systems, vital signs most important to monitor to understand or predict change, and a knowledge of how to develop a good study design. Three levels of methods are presented for the six vital signs of fluvial systems described. Significant experience, time, and money are required for in-depth studies that will likely address several vital signs. Simple, valuable methods, however, can be used to assess all fluvial vital signs even when resources are limited. Initiating a fluvial monitoring program should be done in consultation with an experienced fluvial geomorpholgist. Once a local experience base is built up, a land manager should be able to develop and carry out a wide variety of monitoring studies. If possible, these studies should be done in concert with other monitoring programs, especially those most directly related to stream systems.
The groundwater flow direction is shown by the large blue arrow; the small,yellow arrows point to shallow groundwater wells.
REFERENCES CITED
Abrahams, A.D., Li, G., and Atkinson, J.F., 1995, Step-pool streams: adjustment to maximum flow resistance: Water Resources Research, v. 31, p. 2593–2602, doi: 10.1029/95WR01957.
Allan, J.D., 2004, Influence of land use and landscape setting on the ecological status of rivers: Limnetica, v. 23, p. 187–198
Allmendinger, N.E., Pizzuto, J.E., Potter, N.P., Jr., Johnson, T.E., and Hession, W.C., 2005, The influence of riparian vegetation on stream width, eastern Pennsylvania: Geological Society of America Bulletin, v. 117, p. 229–243, doi: 10.1130/B25447.1.
Andrews, E.D., and Nankervis, J.M., 1995, Effective discharge and the design of channel maintenance fl ows for gravel-bed rivers, in Costa, J.E., Miller, A.J., Potter, K.W., and Wilcock, P.R., eds., Natural and anthropogenic influences in fluvial geomorphology: American Geophysical Union, Geophysical Monograph 89, p. 151–64.
Bedient, P.B., and Huber, W.C., 1988, Hydrology and floodplain: analysis: Addison-Wesley, Reading, Massachusetts, 650 p.
Berger, A.R., 1996, Introduction to geoindicator checklist, in Berger, A.R. and Iams, W.J., eds., Geoindicators: assessing rapid environmental changes in earth systems: Rotterdam, A.A. Balkema, p.383–394.
Berger, A.R., and Iams, W.J., eds., 1996, Geoindicators: assessing rapid environmental changes in earth systems: Rotterdam, A.A. Balkema.
Bridge, J.S., 2003, Rivers and floodplain forms, processes, and sedimentary record: Malden, Blackwell Scientific Publications, 491p.
Brookes, A., 1995, River channel restoration: theory and practice, in Gurnell, A. and Petts, G., eds., Changing river channels: Chichester, John Wiley and Sons, p. 369–388.
Burt, T.P., 1992, The hydrology of headwater catchments, in Calow, P., and Petts, G.E., eds., The Rivers handbook: hydrological and ecological principles: Malden, Blackwell Scientific Publications, v. 1, 526 p.
Bull, W.B., 1997, Discontinuous ephemeral streams: Geomorphology, v. 19, p. 227–276, doi: 10.1016/S0169-555X(97)00016-0.
California Coastal Conservancy, 2001, Watershed management—an overview: southern California wetlands recovery project information station: California Coastal Conservancy, www.wrpinfo.scc.ca.gov/htmls/cc_manage.html, accessed December 2005, 9 p.
Castro, J.M., and Jackson, P.L., 2001, Bankfull discharge recurrence intervals and regional hydraulic geometry relationships: Patterns in the Pacific Northwest, USA: Journal of the American Water Resources Association, v. 37, no. 5, p. 1249–1262, doi: 10.1111/j.1752-1688.2001.tb03636.x.
Chambers, J.C., and Miller, J.R., eds., 2004, Great Basin riparian ecosystems: ecology, management, and restoration: Washington, D.C., Island Press, 303 p.
Chambers, J.C., Tausch, R.J., Korfmacher, J.L., Germanoski, D., Miller, J.R., and Jewett, D.G., 2004, Effects of geomorphic processes and hydrologic regimes of riparian vegetation, in Chambers, J.C., and Miller, J.R., eds., Great Basin riparian ecosystems: ecology, management, and restoration: Washington, D.C., Island Press, p. 196–231.
Chin, A., 1989, Step pools in stream channels: Progress in Physical Geography, v. 13, p. 391–407, doi: 10.1177/030913338901300304.
Church, M., 1992, Channel morphology and typology, in Calow, P., and Petts, G.E., eds., The Rivers handbook: hydrological and ecological principles: Malden, Blackwell Scientific Publications, v. 1, 526 p.
Cooke, R.U., and Doorkamp, J.C., 1990, Geomorphology in environmental management: Oxford, Clarendon Press, 2nd edition, 410 p.
Cottingham, P., Walsh, C., Rooney, G., and Fletcher, T., 2003, Urbanization impacts on stream ecology—from syndrome to cure? in Outcomes of workshops, Symposium on urbanization and stream ecology: Melbourne University, Melbourne, Australia, December 2003, 16 p.
Darby, S.E., and Simon, A., eds., 1999, Incised river channels: processes, forms, engineering and management: Chichester, John Wiley and Sons, 442p.
Davies, T.R., and Sutherland, A.J., 1980, Resistance to flow past deformable boundaries: Earth Surface Processes, v. 5, p. 175–179, doi: 10.1002/esp. 3760050207.
Doyle, M.W., Miller, D.E., and Harbor, J.M., 1999, Should river restoration be based on classification schemes or process models? Insights from the history of geomorphology: ASCE International Conference on Water Resources Engineering, Seattle Washington.
Germanoski, D., and Miller, J.R., 2004, Basin sensitivity to channel incision in response to natural and anthropogenic disturbance, in Chambers, J.C. and Miller, J.R., eds., Great Basin riparian ecosystems: ecology, management, and restoration: Washington, D.C., Island Press, p. 88–123.
Germanoski, D., Miller, J.R., and Latham, D.D., 2002, The importance of event sequencing on the geomorphic impact of wildfire in the central Great Basin: Geological Society of America Abstracts with Programs, v. 34, no. 6, p. 319.
Germanoski, D., and Schumm, S.A., 1993, Changes in braided river morphology resulting from aggradation and degradation: The Journal of Geology, v. 101, p. 451–466.
Gilvear, D.J., and Bryant, R.G., 2003, Analysis of aerial photography and other remotely sensed data, in Kondolf, G.M., and Piégay, H., eds., Tools in fluvial geomorphology: London, Wiley, p. 134–168.
Graf, W., 1983, The arroyo problem—palaeohydrology and palaeohydraulics in the short term, in Gregory, K.L., ed., Background to palaeohydrology; a perspective: New York, John Wiley and Sons, 486 p.
Grant, G.E., Swanson, F.J., and Wolman, M.G., 1990, Pattern and origin of stepped bed morphology in high-gradient streams, Western Cascades, Oregon: Geological Society of America Bulletin, v. 102, p. 340–352, doi: 10.1130/0016-7606(1990)102<0340:PAOOSB>2.3.CO;2.
Gregory, K.J., and Walling, D.E., eds., 1987, Human activity and environmental processes: Chichester, John Wiley and Sons, 466 p.
Hall, S.A., 1990, Channel trenching and climate change in the southern U.S. Great Plains: Geology, v. 18, p. 342–345, doi: 10.1130/0091-7613(1990) 018<0342:CTACCI>2.3.CO;2.
Jewett, D.G., Lord, M.L., Miller, J.R., and Chambers, J.C., 2004, Geomorphic and hydrologic controls on surface and subsurface fl ow regimes in riparian meadow ecosystems, in Chambers, J.C., and Miller, J.R., eds., Great Basin riparian ecosystems: ecology, management, and restoration: Washington, D. C., Island Press, p. 124–161.
Juracek, K.E., and Fitzpatrick, F.A., 2003, Limitations and implications of stream classification: Journal of the American Water Resources Association, v. 39, p. 659–670, doi: 10.1111/j.1752-1688.2003.tb03683.x.
Keller, E.A., and Melhorn, W.N., 1978, Rhythmic spacing and origin of pools and riffles: Geological Society of America Bulletin, v. 89, p. 723–730, doi: 10.1130/0016-7606(1978)89<723:RSAOOP>2.0.CO;2.
Keller, E.A., and Swanson, F.J., 1979, Effects of large organic material on channel form and fluvial processes: Earth Surface Processes, v. 4, p. 361–380, doi: 10.1002/esp.3290040406.
Knighton, D., 1998, Fluvial forms and processes: a new perspective: London and New York, Copublished by Arnold and Oxford University Press, 383 p.
Kochel, R.C., Miller, J.R., Lord, M.L., and Martin, T., 2005, Geomorphic problems with in-stream structures using natural channel design strategy for stream restoration projects in North Carolina: Geological Society of America Abstracts with Programs, v. 37, no. 7, p. 329.
Kondolf, G.M., Piégay, H., and Sear, D., 2003, Integrating geomorphologic tools in ecological and management studies, in Kondolf, G.M., and Piégay, H., eds., Tools in Fluvial Geomorphology: Chichester, John Wiley and Sons, 688 p.
Kondolf, G.M., and Piégay, H., eds., 2003, Tools in Fluvial Geomorphology: Chichester, John Wiley and Sons, 688 p.
Langbein, W.B., and Schumm, S.A., 1958, Yield of sediment in relation to mean annual precipitation: American Geophysical Union Transactions, v. 39, p. 1076–1084.
Lane, E.W., 1955, The importance of fl uvial morphology in hydraulic engineering: American Society of Civil Engineering Proceedings, v. 81, paper 745, p. 1–17.
Leopold, L.B., 1994, A view of the river: Cambridge, Massachusetts, Harvard University Press, 298 p.
McClintlock, K.A., Harbor, J.A., and Wilson, T.P., 1995, Assessing the hydrologic impact of land use change in wetland watersheds: a case study from northern Ohio, USA, in McGregor, D.F.M., and Thompson, D.A., eds., Geomorphology and Land Management in a Changing Environment: Chichester, U.K., John Wiley and Sons, p. 107–119.
Miller, J.R., and Ritter, J.B., 1996, An examination of the Rosgen classification of natural rivers: Catena, v. 27, p. 295–299.
Miller, J.R., House, K., Germanoski, D., Tausch, R.J., and Chambers, J.C., 2004, Fluvial geomorphic responses to Holocene climatic change, in Chambers, J.C., and Miller, J.R., eds., Great Basin riparian ecosystems: ecology, management, and restoration: Washington, D. C., Island Press, p. 49–87.
Miller, J.R., Lord, M.L., Yurkovich, S., Mackin, G., and Kolenbrander, L., 2005, Historical trends in sedimentation rates and sediment provenance, Fairfield Lake, western North Carolina: Journal of the American Water Resources Association, v. 41, p. 1053–1075, doi: 10.1111/j.1752-1688. 2005.tb03785.x.
Mollard, J.D., 1973, Air photo interpretation of fluvial features: Proceedings of the 7th Canadian Hydrology Symposium, v.6, no.4, p. 341–380.
Osterkamp, W.R., and Schumm, S.A., 1996, Geoindicators for river and river valley
monitoring, in Berger, A.R., and Iams, W.J., eds., Geoindicators: assessing rapid environmental changes in earth systems: Rotterdam, A.A. Balkema, p. 83–100.
Petts, G.E., and Amoros, C., 1996. Fluvial hydrosystems: London, Chapman and Hall, 336 p.
Richards, K., 1982, Rivers: form and process in alluvial channels: London, Methuen, 360p.
Reid, L.M., and Dunne, T., 1996, Rapid evaluation of sediment budgets: Catena Verlag, Reiskirchen, Germany, 164 p.
Ritter, D.F., Kochel, R.C., and Miller, J.R., 2002, Process geomorphology (fourth edition): New York, McGraw Hill, 560 p.
Rosgen, D.L., 1996, Applied river morphology (second edition): Pagosa Springs, Colorado, Wildland Hydrology, 390 p.
Sanders, L.L., 1998, A manual of fi eld hydrogeology: Upper Saddle River, Prentice Hall, Inc., 381 p.
Schumm, S.A., 1977, The fl uvial system: New York, John Wiley and Sons, 338 p.
Schumm, S.A., 1981, Evolution and response of the fl uvial system, sedimentologic implications: SEPM Special Publication No. 31, p. 19–29.
Schumm, S.A., 1985, Patterns of alluvial rivers: Annual Review of Earth and Planetary Sciences 13: p. 5–27.
Schumm, S.A., 1991, To Interpret the Earth: Ten Ways to Be Wrong: Cambridge, Cambridge University Press, 180 p.
Schumm, S.A., Harvey, M.D., and Watson, C.C., 1984, Incised channels: morphology, dynamics, and control: Littleton, Colorado, Water Resources Publications, 200 p.
U.S. Environmental Protection Agency, 2002, Urbanization and streams: studies of hydrologic impacts: U.S. EPA, Offi ce of Wetlands, Oceans, andWatersheds. ww.epa.gov/owow/NPS/urbanize/report.html, accessed December 2005.
Waters, M.R., and Haynes, C.V., 2001, Late Quaternary arroyo formation and climate change in the American Southwest: Geology, v. 29, no. 5, p. 399–402, doi: 10.1130/0091-7613(2001)029<0399:LQAFAC>2.0.CO;2.
Whitaker, J.G., 1987, Sediment transport in step-pool streams, in Thorne, C.R., Bathurst, J.C., and Hey, R.D., eds., Sediment transport in gravel-bed rivers. Chichester, John Wiley and Sons, p. 545–570.
Williams, G.P., 1978, Bankfull discharge of rivers: Water Resources Research, v. 14, p. 1141–1158, doi: 10.1029/WR014i006p01141.
Wohl, E.E., and Grodek, T., 1994, Channel bed-steps along Nahal Yael, Negev Desert, Israel: Geomorphology, v. 9, p. 117–126, doi: 10.1016/0169- 555X(94)90070-1.
Wolman, M.G., 1967, A cycle of sedimentation and erosion in urban river channels: Geografiska Annaler, v. 49-A, p. 385–395, doi: 10.2307/520904.
Wolman, M.G., and Gerson, R., 1978, Relative scales of time and effectiveness of climate in watershed geomorphology: Earth Surface Processes, v. 3, p. 189–208, doi: 10.1002/esp.3290030207.
ADDITIONAL RESOURCES FOR METHODS GROUPED BY VITAL SIGN
General
Agents of Watershed Change, Online Training in Watershed Management, U.S. Environmental Protection Agency: www.usepa.gov.
Allen, P.A., 1997, Earth Surface Processes: Malden, Blackwell Science Ltd., 404 p.
Geoindicators Initiative, International Union of Geological Sciences: www.lgt.lt/geoin/.
Geologic and Hydrologic Monitoring Parameters, National Park Service: www.nps.gov.
Kondolf, G.M., and Piégay, H., eds., 2003, Tools in Fluvial Geomorphology: Chichester, John Wiley and Sons, 688 p.
Office of Surface Water, U.S. Geological Survey: water.usgs.gov.
Schumm, S.A., and Winkley, B.R., eds., 1994, The variability of large alluvial rivers: New York: American Society of Civil Engineers Press, 459 p.
Stream Systems Technology Center, U.S. Forest Service: www.stream.fs.fed.us.
Water Science Glossary of Terms, U.S. Geological Survey, water.usgs.gov.
1. Watershed Landscape
Applied Fluvial Geomorphology Ad Hoc Committee, 2004, Position Statement on Applied Fluvial Geomorphology: Quaternary Geology and Geomorphology Division, Geological Society of America, www.geo.wvu.edu/~kite/QG&GFluvGeomPositionStatement22March2004.html, accessed December 2005.
Arnold, C.L., Boison, P.J., and Patton, P.C., 1982, Sawmill Brook: an example of rapid geomorphic change related to urbanization: The Journal of Geology, v. 90, p. 155–166.
Clark, J.J., and Wilcock, P.R., 2000, Effects of land-use change on channel morphology in northeastern Puerto Rico: Geological Society of America Bulletin, v. 112, no. 12, p. 1763–1777, doi: 10.1130/0016-7606(2000)11 2<1763:EOLUCO>2.0.CO;2.
Costa, J.E., 1975, Effects of agriculture on erosion and sedimentation in the Piedmont Province, Maryland: Geological Society of America Bulletin, v. 86, p. 1281–1286, doi: 10.1130/0016-7606(1975)86<1281:EOAOEA>2.0.CO;2.
Davies-Colley, R.J., 1997, Stream channels are narrower in pasture than in forest: New Zealand Journal of Marine and Freshwater Research, v. 31, p. 599–608.
Hupp, C.R., and Osterkamp, W.R., 1996, Riparian vegetation and fluvial geomorphic processes: Geomorphology, v. 14, p. 277–295, doi: 10.1016/0169-555X(95)00042-4.
Kuhnle, R.A., Bingner, R.L., Foster, G.R., et al., 1996, Effect of land use changes on sediment transport in Goodwin Creek: Water Resources Research, v. 32, no. 10, p. 3189–3196, doi: 10.1029/96WR02104.
Liebault, F., and Piégay, H., 2001, Assessment of channel changes due to longterm bedload supply decrease, Roubion River, France: Geomorphology, v. 36, no. 3–4, p. 167–186, doi: 10.1016/S0169-555X(00)00044-1.
Schumm, S.A., 1969, River metamorphosis: Journal of the Hydraulics Division: Proceedings of the American Society of Civil Engineers, v. HY1, p. 253–275.
Trimble, S.W., 1981, Changes in sediment storage in the Coon Creek Basin, Driftless Area, Wisconsin, 1853 to 1975: Science, v. 214, p. 181–183, doi: 10.1126/science.214.4517.181.
Trimble, S.W., 1999, Decreased rates of alluvial sediment storage in the Coon Creek Basin, Wisconsin, 1975–1993: Science, v. 285, p. 1244–1246, doi: 10.1126/science.285.5431.1244.
Whiting, P.J., and Bradley, J.B., 1993, A process-based classifi cation for headwater streams: Earth Surface Processes and Landforms, v. 18, p. 603–612, doi: 10.1002/esp.3290180704.
Wolman, W.G., and Riggs, editors, H.C., 1990, Surface water hydrology: Boulder, Colorado: Geological Society of America, Geology of North America, Volume O-1, 374 p.
Wolman, M.G., and Schick, A.P., 1967, Effects of construction on fl uvial sediment, urban and suburban areas of Maryland: Water Resources Research, v. 3, no. 2, p. 451–464, doi: 10.1029/WR003i002p00451.
Yorke, T.H., and Herb, W.J., 1978, Effects of urbanization on streamflow and sediment transport in the Rock Creek and Anacostia River Basins, Montgomery County, Maryland, 1962–1974: U.S. Geological Survey Professional Paper 1003, 71 p.
2. Hydrology
Baker, V.R., Kochel, R.C., and Patton, P.C., 1988, Flood geomorphology: New York, John Wiley and Sons, 503 p.
deMarsily, G., 1986, Quantitative hydrology: New York, Academic Press, 564 p.
Dunne, T., and Leopold, L.B., 1978, Water in Environmental Planning: San Francisco, W.H. Freeman, 818 p.
Edmunds, W.M., 1996, Indicators in the groundwater environment of rapid environmental change, in Berger, A.R. and Iams, W.J., eds., Geoindicators: assessing rapid environmental changes in earth systems: Rotterdam, A.A. Balkema, p. 121–136.
Freeze, R.D., and Cherry, J.A., 1979, Groundwater: Englewood Cliffs, New Jersey, Prentice-Hall, 604 p.
Gomez, B., Mertes, L.A.K., Phillips, J.D., Magilligan, F.J., and James, L.A., 1995, Sediment characteristics of an extreme fl ood: 1993 upper Mississippi River Valley: Geology, v. 23, no. 11, p. 963–966, doi: 10.1130/0091-7613(1995)023<0963:SCOAEF>2.3.CO;2.
Gupta, A., and Fox, H., 1974, Effects of high-magnitude floods on channel form: a case study in Maryland Piedmont: Water Resources Research, v. 10, no. 3, p. 499–509, doi: 10.1029/WR010i003p00499.
Lins, H.F., and Slack, J.R., 1999, Streamfl ow trends in the United States: Geophysical Research Letters, v. 26, no. 2, p. 227–230, doi: 10.1029/1998GL900291.
Mosley, P.M., 1983, Flow requirements for recreation and wildlife in New Zealand Rivers—a review: Journal of Hydrology (Amsterdam), v. 22, no. 2, p. 152–174.
Mosley, P.M., 1985, River channel inventory, habitat and instream flow assessment: Progress in Physical Geography, v. 9, p. 494–523, doi: 10.1177/030913338500900402.
Osterkamp, W.R., and Hedman, E.R., 1982, Perennial-streamfl ow characteristics related to channel geometry and sediment in Missouri River basin: U.S. Geological Survey Professional Paper 1241.
Pizzuto, J.E., 1994, Channel adjustments to changing discharges, Powder River, Montana: Geological Society of America Bulletin, v. 106, no. 11, p. 1494–1501, doi: 10.1130/0016-7606(1994)106<1494:CATCDP>2.3.CO;2.
Price, M., 1985, Introducing groundwater: London, Allen and Unwin, 301 p.
Stall, J.B., and Herricks, E.E., 1982, Evaluating aquatic habitat using stream network structure and streamfl ow predictions, in Craig, R.G., and Craft, J.L., Applied Geomorphology: London, George Allen and Unwin, p. 240–253.
Williams, G.P., 1978, Bank-full discharge of rivers: Water Resources Research, v. 14, no. 6, p. 1141–1153, doi: 10.1029/WR014i006p01141.
3. Sediment Transport
Guy, H.P., and Norman, V.W., 1970, Field methods for measurement of fluvial sediment: U.S. Geological Survey, Techniques of Water Resources Investigation, Book 3, Chapter C-2.
Habersack, H.M., and Laronne, J.B., 2001, Bed load texture in an alpine gravel bed river: Water Resources Research, v. 37, no. 12, p. 3359–3370, doi:10.1029/2001WR000260.
Lane, E.W., 1955, The importance of fl uvial morphology in hydraulic engineering: American Society of Civil Engineering Proceedings, v. 81, paper 745,p. 1–17.
Lisle, T.E., Iseya, F., and Ikeda, H., 1993, Response of a channel with alternate bars to a decrease in supply of bed load: a fl ume experiment: Water Resources Research, v. 29, no. 11, p. 3623–3629, doi: 10.1029/93WR01673.
Milliman, J.D., and Meade, R.H., 1983, World-wide delivery of river sediment to the oceans: The Journal of Geology, v. 91, no. 1, p. 1–21.
Miller, D.J., and Benda, L.E., 2000, Effects of punctuated sediment supply on valley-floor landforms and sediment transport: Geological Society of America Bulletin, v. 112, no. 12, p. 1814–1824, doi: 10.1130/0016-7606 (2000)112<1814:EOPSSO>2.0.CO;2.
Phillips, J.D., 1991, Fluvial sediment delivery to a coastal plain estuary in the Atlantic Drainage of the United States: Marine Geology, v. 98, p. 121–134, doi: 10.1016/0025-3227(91)90040-B.
Rice, S., and Church, M., 1996, Sampling surfi cial gravels: the precision of size distribution percentile estimates: Journal of Sedimentary Research, v. 66, no. 3, p. 654–665.
Royall, D., 2000, Lake-sediment-based evaluation of recent sediment yield from a small Appalachian watershed, Thompson Lake, Virginia: Physical Geography, v. 21, no. 1, p. 66–88.
Sterling, S.M., and Church, M., 2002, Sediment trapping characteristics of a pit trap and the Helley-Smith sampler in a cobble gravel bed river: Water Resources Research, v. 38, no. 8, p. 19-1–19-11.
Sutherland, D.G., and Ball, M.H., 2002, Evolution of a landslide-induced sediment wave in the Navarro River, California: Geological Society of America Bulletin, v. 114, no. 8, p. 1036–1048, doi: 10.1130/0016-7606(2002) 114<1036:EOALIS>2.0.CO;2.
Thompson, D.M., 1995, The effects of large organic debris on sediment processes and stream morphology in Vermont: Geomorphology, v. 11, p. 235–244, doi: 10.1016/0169-555X(94)00064-X.
Trimble, S.W., 1983, A sediment budget for Coon Creek Basin in the Driftless Area, Wisconsin, 1853–1977: American Journal of Science, v. 283, p. 454–474.
Vanoni, V.A., ed., 1975, Sedimentation engineering: New York, American Society of Civil Engineering Press, 424 p.
Visher, G.S., 1969, Grain size distributions and depositional processes: Journal of Sedimentary Petrology, v. 39, no. 3, p. 1074–1106.
Wathen, S.J., and Hoey, T.B., 1998, Morphological controls on the downstream passage of a sediment wave in a gravel-bed stream: Earth Surface Processes and Landforms, v. 23, p. 715–730, doi: 10.1002/(SICI)1096-9837(199808)23:8<715::AID-ESP877>3.0.CO;2-0.
Wilcock, P.R., 1993, Critical shear stress of natural sediments: Journal of Hydraulic Engineering, v. 119, no. 4, p. 491–505, doi: 10.1061/(ASCE)0733-9429(1993)119:4(491).
Wolman, M.G., 1954, A method of sampling coarse river-bed material: American Geophysical Union Transactions, v. 35, no. 6, p. 951–956.
4. Channel: Cross-section
Allred, T.M., and Schmidt, J.C., 1999, Channel narrowing by vertical accretion along the Green River near Green River, Utah: Geological Society of America Bulletin, v. 111, no. 12, p. 1757–1772, doi: 10.1130/0016-7606 (1999)111<1757:CNBVAA>2.3.CO;2.
Bull, L.J., 1997, Magnitude and variation in the contribution of bank erosion to the suspended sediment load of the River Severn, UK: Earth Surface Processes and Landforms, v. 22, p. 1109–1123, doi: 10.1002/(SICI)1096-9837(199712)22:12<1109::AID-ESP810>3.0.CO;2-O.
Dunaway, D., Swanson, R.S., Wendel, J., and Clary, W., 1994, The effect of herbaceous plant communities and soil textures on particle erosion of alluvial streambanks: Geomorphology, v. 9, p. 47–56, doi: 10.1016/0169-555X(94)90030-2.
Millar, R.G., and Quick, M.C., 1998, Stable width and depth of gravel-bed rivers with cohesive banks: Journal of Hydraulic Engineering, v. 124, no. 10, p. 1005–1013, doi: 10.1061/(ASCE)0733-9429(1998)124:10(1005).
Millar, R.G., 2000, Infl uence of bank vegetation on alluvial channel patterns: Water Resources Journal, v. 205, p. 59–75.
Parker, G., 1979, Hydraulic geometry of active gravel rivers: Journal of the ASCE Hydraulics Division, v. HY9, p. 1185–1201.
Thorne, C.R., 1998, River width adjustment. I: Processes and mechanisms: Journal of Hydraulic Engineering, v. 124, no. 9, p. 881–902, doi: 10.1061/(ASCE)0733-9429(1998)124:9(881).
Tooth, S., and McCarthy, T.S., 2002, Geological controls on the formation of alluvial meanders and floodplain wetlands; the example of the Klip River, eastern Free State, South Africa: Earth Surface Processes and Landforms, v. 27, no. 8, p. 797–815, doi: 10.1002/esp.353.
5. Channel: Planform
Chang, H.H., 1988, Fluvial processes in river engineering. New York: John Wiley and Sons. Gran, K., and Paola, C., 2001, Riparian vegetation controls on braided stream dynamics: Water Resources Research, v. 37, no. 12, p. 3275–3283, doi:10.1029/2000WR000203.
Gurnell, A.M., Petts, G.E., Hannah, D.M., Smith, B.P.G., Edwards, P.J., Kollmann, J., Ward, J.V., and Tockner, K., 2001, Riparian vegetation and island formation along the gravel-bed Fiume Taliamento, Italy: Earth Surface Processes and Landforms, v. 26, p. 31–62, doi: 10.1002/1096-9837(200101)26:1<31::AID-ESP155>3.0.CO;2-Y.
Lewin, J., 1976, Initiation of bedforms and meanders in coarse-grained sediment: Geological Society of America Bulletin, v. 87, p. 281–285, doi: 10. 1130/0016-7606(1976)87<281:IOBFAM>2.0.CO;2.
Malakoff, D., 2004, News focus, profile: Dave Rosgen: the river doctor: Science, v. 305, no. 5686, p. 937–939, doi: 10.1126/science.305.5686.937.
Osterkamp, W.R., 1998, Processes of fl uvial island formation, with examples from Plum Creek, Colorado and Snake River, Idaho: Wetlands, v. 18, no. 4, p. 530–545.
Power, M.E., Parker, G., Dietrich, W.E., and Sun, A., 1995, How does floodplain width affect floodplain river ecology? A preliminary exploration using simulations: Geomorphology, v. 13, p. 301–317, doi: 10.1016/0169-555X(95)00039-8.
Rhoads, B.L., and Welford, M.R., 1991, Initiation of river meandering: Progress in Physical Geography, v. 15, no. 2, p. 127–156, doi: 10.1177/030913339101500201.
Rosgen, D.L., 1994, A classifi cation of natural rivers: Catena, v. 22, p. 169–199, doi: 10.1016/0341-8162(94)90001-9.
Stolum, H.H., 1998, Planform geometry and dynamics of meandering rivers: Geological Society of America Bulletin, v. 110, no. 11, p. 1485–1498, doi: 10.1130/0016-7606(1998)110<1485:PGADOM>2.3.CO;2.
6. Channel: Longitudinal Profile
Chang, H.H., 1980, Geometry of gravel streams: Journal of the Hydraulics Division: Proceedings of the American Society of Civil Engineers, v. 106, n. HY9, p. 1443–1456.
Gardiner, T., 1983, Some factors promoting channel bank erosion, River Lagan: County Down: Journal of Earth Sciences, v. 5, no. 2, p. 231–239.
Grant, G.E., Swanson, F.J., and Wolman, M.G., 1990, Pattern and origin of stepped-bed morphology in high-gradient streams, Western Cascades, Oregon: Geological Society of America Bulletin, v. 102, p. 340–352, doi: 10.1130/0016-7606(1990)102<0340:PAOOSB>2.3.CO;2.
Kirby, E., and Whipple, K., 2001, Quantifying differential rock-uplift rates via stream profile analysis: Geology, v. 29, no. 5, p. 415–418, doi: 10.1130/0091-7613(2001)029<0415:QDRURV>2.0.CO;2.
Lisle, T.E., 1982, Effects of aggradation and degradation on riffle-pool morphology in natural gravel channels, north California: Water Resources Research, v. 18, no. 6, p. 1643–1651, doi: 10.1029/WR018i006p01643.
Montgomery, D.R., and Buffi ngton, J.M., 1997, Channel-reach morphology in mountain drainage basins: Geological Society of America Bulletin, v. 109, no. 5, p. 596–611, doi: 10.1130/0016-7606(1997)109<0596: CRMIMD>2.3.CO;2.
Montgomery, D.R., Abbe, T.B., Peterson, N.P., Buffi ngton, J.M., Schmidt, K.M., and Stock, J.D., 1996, Distribution of bedrock and alluvial channels in forested mountain drainage basins: Nature, v. 381, p. 587–589, doi: 10.1038/381587a0.
Reed, J.C., 1981, Disequilibrium profi le of the Potomac River near Washington, D.C.—A result of lowered base level or Quaternary tectonics along the Fall Line?: Geology, v. 9, p. 445–450, doi: 10.1130/0091-7613(1981)9<4 45:DPOTPR>2.0.CO;2.
Shimizu, Y., and Itakura, T., 1989, Calculation of bed variation in alluvial channels: Journal of Hydraulic Engineering, v. 115, no. 3, p. 367–385.
Sinha, S.K., and Parker, G., 1996, Causes of concavity in longitudinal profiles of rivers: Water Resources Research, v. 32, no. 5, p. 1417–1428, doi: 10.1029/95WR03819.
Thompson, D.M., Nelson, J.M., and Wohl, E.E., 1998, Interactions between pool geometry and hydraulics: Water Resources Research, v. 34, no. 12, p. 3673–3681, doi: 10.1029/1998WR900004.
Trimble, S.W., 1977, The fallacy of stream equilibrium in contemporary denudation studies: American Journal of Science, v. 277, p. 876–887.
Wolman, M.G., 1987, Sediment movement and knickpoint behavior in a small Piedmont drainage basin: Geografiska Annaler, v. 69A, p. 5–14, doi: 10.2307/521362.