Introduction
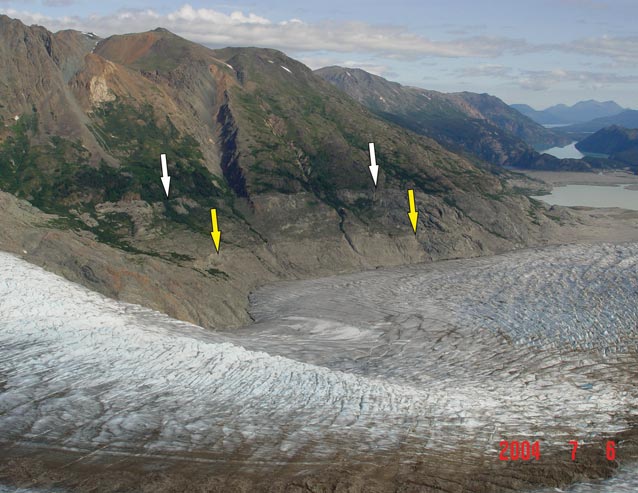
Alaska’s Southeast parks are among the most impressive in the world. Cold lofty peaks, valley glaciers, and ice fields back verdant Sitka spruce forests along the fjord-incised coast, with forests of the smaller white spruce inland. The parks are situated on the Pacific Rim of Fire, a necklace of volcanoes and earthquake-prone ground at the margin of the Pacific Plate. Earthquakes, active volcanoes, and steep slopes are the ingredients for large landslides, and river floods and tsunami are frequent in this landscape. The coastal region is battered by Pacific storms, which further act on these slopes, priming and conditioning them for failure over decades, centuries, and millennia.
Although climatically diverse, Alaska is likely to experience an overall increase in temperatures through this century, especially in winter, and winters are likely to become wetter and summers drier (USGRP 2009). Landscape responses to these climate changes will be complex. Increased snow loads can influence glacier dynamics, trigger landslides during rapid melt, and cause flooding. Intense rainstorms can trigger shallow landslides.
Two longer term consequences of climate warming that will affect slope stability are glacier thinning and permafrost degradation. Changes to glaciers are easy to recognize. Trim lines and lateral moraines benchmark the limits of previous larger extents (Figure 1). Time lapse photography and spectacular videos of calving glaciers into tidewater attest to the dynamic nature of glaciers as slow rivers of ice. Permafrost degradation is less obvious, but more insidious. You cannot tell just by looking at a steep rock face whether or not permafrost is present.
Influence of glaciers on slope stability
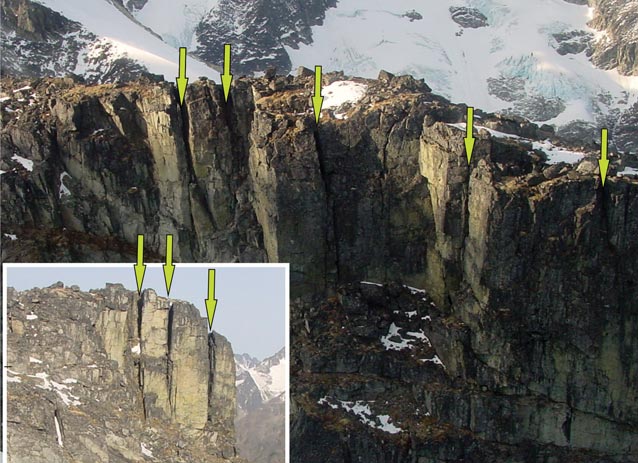
Marten Geertsema
Glaciers have strong effects on the stability of slopes. They erode, redistribute and deposit soil. Sediment exposed during glacier retreat is temporarily unvegetated and often draped over steep slopes and thus vulnerable to debris slides and flows (Huggel et al. 2012).
Valley glaciers condition rock masses for failure in four main ways (Geertsema and Chiarle 2013). First, they erode and deepen valleys and steepen valley walls. Second, flowing glacier ice exerts stresses on valley floors and walls and can fracture bedrock. Third, as glaciers thin and retreat, support is removed—slopes are ‘debutressed’. Fourth, relaxation of glacier-induced stresses results in the widening of fractures and joints, conditioning the slopes for mass movement (Figure 2).
Glaciers that hang on steep rock faces and firn or ice on ridges and summits are potential sources of ice avalanches; they also affect the stability of the underlying rock (Fischer et al. 2013). A reduction in the extent of snow and ice on steep slopes at high elevation exposes fresh rock to strong temperature cycles. Mechanical loading due to more and wetter seasonal snow fall may also destabilize some steep alpine slopes. Enhanced warming of firn and alpine permafrost in a warmer climate is another destabilizing influence (see below). More meltwater will reach potential failure surfaces via fractures and clefts in rock.
We recognize three main categories of mass movement on glacially conditioned rock slopes: rock fall, deep-seated slope deformation, and catastrophic rock avalanches. Rock fall rates are high on steep slopes in fractured rock and on recently deglaciated cirque headwalls (Fischer et al. 2013). Rates are lower on slopes that have been exposed for longer periods. Glacial debuttressing since the Little Ice Age may also cause deep-seated rock sagging in mountains, a process known as “sackung”. Slow sagging of rock slopes may continue indefinitely, for centuries or millennia, but in some instances can lead to catastrophic slope failure. A large, slowly sagging slope (Figure 3) in Glacier Bay National Park has been studied by Wieczorek et al. (2007). If it were to fail catastrophically, the rock mass would enter the Tidal Inlet and produce a large and potentially destructive displacement wave. Glacial debuttressing may also be one of the factors responsible for large-volume, long-runout landslides known as rock avalanches, which are characterized by a streaming behavior. A combined rock and ice avalanche from Lituya Mountain in 2012 travelled more than 5 miles (8 km) over a glacier (Figure 4; Geertsema 2012).
Outburst floods from lakes dammed by glaciers and moraines
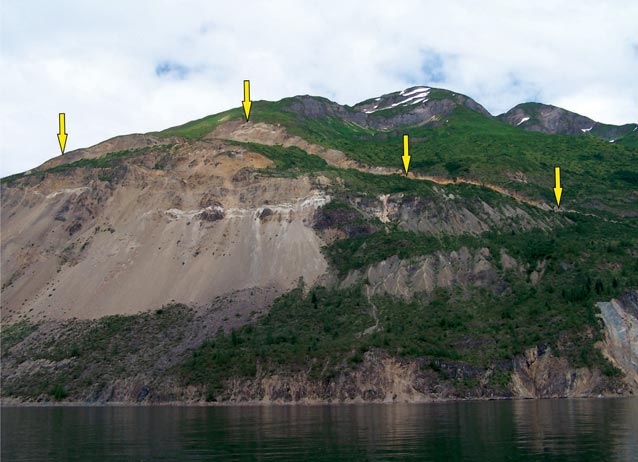
NPS Photo
Many glaciers in Alaska parks impound large bodies of water that may drain suddenly, causing downvalley floods, termed jökulhlaups (Figure 5). These floods are far larger than normal nival and rainfall-triggered floods (Costa and Schuster 1988; Clague and Evans 1994; Loso et al., this volume). The water bodies exist on top of, within, beneath, or at the margins of glaciers. Those are the margins of alpine glaciers are commonly the most prone to draining, especially if located in trunk valleys at the toes of glaciers flowing out of tributary valleys.
Glacial lakes may drain suddenly and unexpectedly following a long period of stability due to progressive wastage of the glacier dam (Costa and Schuster 1988; Clague and Evans, 1994). Sudden draining of these lakes typically happens due to rapid development of subglacial channels that serve as conduits for outflowing water. Glacier dams may also fail by collapse following rapid glacier advances (surges) that block streams for only months.
Lakes also developed behind Little Ice Age end moraines as glaciers retreated in the late 19th and early 20th centuries (Costa and Schuster 1988; O’Connor and Costa 1993; Clague and Evans 1994, 2000). Many of these moraine dams are unstable and vulnerable to failure (Figure 6) because they are steep-sided and consist of loose sediment. Irreversible rapid incision of the dam may be caused by a large overflow triggered by an ice avalanche or a rock fall. Other failure mechanisms include earthquakes, slow melt of buried ice, and removal of fine sediment from the dam (piping).
Floods resulting from failures of glacier and moraine dams may transform into debris flows as they travel down steep valleys (Clague and Evans 2000). Entrainment of sediment and woody plant debris by floodwaters may cause peak discharge to increase downvalley, with a potential increase in travel distance and destructiveness.
Outburst floods from lakes dammed by glaciers and moraines erode, transport, and deposit huge amounts of sediment over distances of tens of kilometers. They alter river floodplains tens of kilometers from the flood source. They broaden floodplains, destroy pre-flood channels, and create a new multi-channel, braided planform. The changes can persist for decades after the flood, although rivers quickly reestablish their pre-flood grades by incising the flood deposits.
Degradation of mountain permafrost
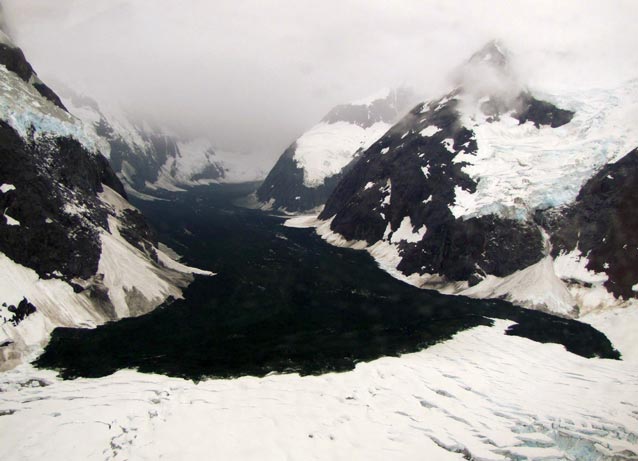
Drake Olson
Permafrost is widespread in Alaska, both at lower elevations in the northern part of the state and in high mountains. Periglacial features such as stone stripes, rock glaciers, solifluction lobes, and palsas attest to its presence. It is now recognized that the degradation of mountain permafrost in our warming climate is contributing to an increase in landslides (Geertsema et al. 2006; Gruber and Haeberli 2007; Huggel et al. 2008, 2012). The influence of permafrost on rock instability involves many processes, including fracturing and cleft widening by the formation of ice, strength reduction by thawing and water percolation, and perhaps resealing by surface freezing (Gruber and Haeberli 2007; Hasler et al. 2012).
Three very large rock-ice avalanches have occurred on steep and rather cold bedrock slopes in the St. Elias Mountains within the past decade (Figure 4, 7; Huggel et al. 2008, Lipovsky et al. 2008; Geertsema 2012). The Mount Steller, Alaska event occurred at the end of a particularly warm summer (2005); the Mt. Steele, Yukon (2007) and Mount Lituya, Alaska (2012) events seem not to be directly related to a particularly warm summer or year. Huggel et al. (2008) modelled the thermal regime of Mount Steller, which highlighted a potential warming effect on underlying rock of the ice covering the summit. High summit glaciers such as the one on Mount Steller, may play a role in catastrophic rock-ice avalanches in other cold mountain ranges (Huggel et al. 2012).
Interactions between ice cover and underlying permafrost are diverse and complex. Nevertheless, enhanced warming of permafrost and the entry of meltwater into rock fractures are likely to be involved, at least to some extent, in the three events mentioned above. Firn temperatures in cold areas are strongly dependent on the amount of meltwater (Hoelzle et al. 2011). An increase in melt during the warm period 1990 to 2005 (Pleasant Camp climate station, Environment Canada) likely led to a gradual warming of the ice cover.If this meltwater reached the underlying permafrost it could reduce rock strength along discontinuities in the bedrock (Gruber and Haeberli 2007; Hasler et al. 2012).
Event chains
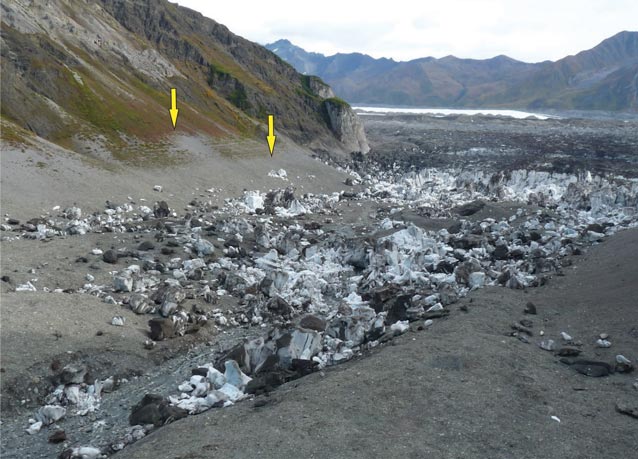
NPS Photo
Geomorphic events rarely act in isolation. A rockslide can transform into a rock avalanche or debris flow at the base of a steep slope if it encounters water-saturated soils, snow, or glacier ice. A landslide can also impound a stream, producing upstream flooding or a downvalley flood if the dam fails. Flooding from glacial outburst floods can erode stream banks and trigger landslides and debris flows. Landslides can generate large displacement waves such as the 1700-foot wave in Lituya Bay in 1958 (Miller 1960).
Conclusions
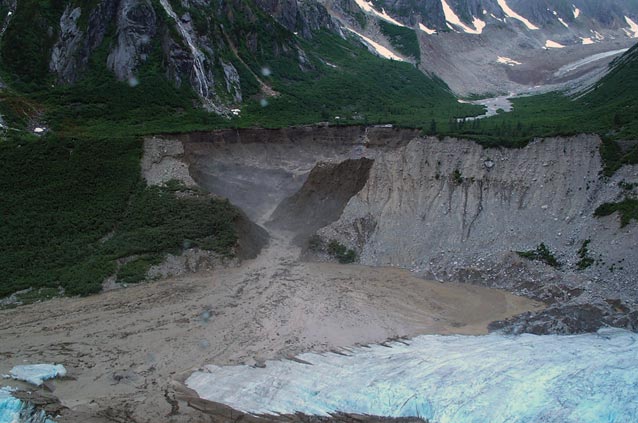
TEMSCO Helicopters, Inc.
Alaska’s parks are dynamic and are undergoing constant geomorphic change as glaciers and streams erode and cliffs collapse. Based on climate projections, some permafrost in Alaska will thaw, and many glaciers will thin and retreat, over the remainder of this century, uncovering potentially unstable valley walls. Both permafrost thaw and glacier thinning will contribute to an increase in the incidence of landslides.
Part of a series of articles titled Alaska Park Science - Volume 12 Issue 2: Climate Change in Alaska's National Parks.
Last updated: October 26, 2021