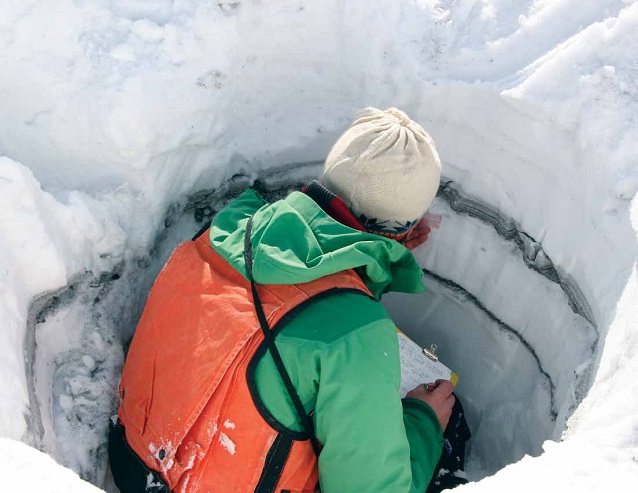
USGS photograph by A. Diefenbach
Introduction
Alaska is one of the most vigorously volcanic regions on the planet, and Alaska’s national parks are home to many of the state’s most active volcanoes. These pose both local and more distant hazards in the form of lava and pyroclastic flows, lahars (mudflows), ash clouds, and ash fall. Alaska’s volcanoes lie along the arc of the Aleutian-Alaskan subduction zone, caused as the oceanic Pacific plate moves northward and dips below the North American plate. These volcanoes form as water-rich fluid from the down-going Pacific plate is released, lowering the melting temperature of rock in the overlying mantle and enabling it to partially melt. The melted rock (magma) migrates upward, collecting at the base of the approximately 25 mile (40 km) thick crust, occasionally ascending into the shallow crust, and sometimes erupting at the earth’s surface.
During volcanic unrest, scientists use geophysical signals to remotely visualize volcanic processes, such as movement of magma in the upper crust. In addition, erupted volcanic rocks, which are quenched samples of magmas, can tell us about subsurface magma characteris-tics, history, and the processes that drive eruptions. The chemical compositions of and the minerals present in the erupted magmas can reveal conditions under which these magmas were stored in crustal “chambers”. Studies of the products of recent eruptions of Novarupta (1912), Aniakchak (1931), Trident (1953-74), and Redoubt (2009) volcanoes reveal the depths and temperatures of magma storage, and tell of complex interactions between magmas of different compositions. One goal of volcanology is to determine the processes that drive or trigger eruptions. Information recorded in the rocks tells us about these processes. Here, we demonstrate how geologists gain these insights through case studies from four recent eruptions of volcanoes in Alaska national parks.
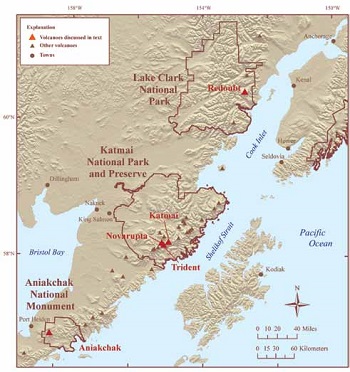
Methods
Investigation of the magmatic processes beneath volcanoes starts with field study of the erupted deposits. For recent eruptions, volcanologists use photographs, satellite images, seismic data, and field observations to correlate deposits with individual explosions or other types of activity such as lava-dome growth. For older eruptions, we use the order and distribution of layered deposits (stratigraphy), such as those from ash falls and pyroclastic flows, to ascertain the sequence of events.
Volcanic rocks typically contain a few to nearly 50% relatively large crystals of rock-forming minerals (phenocrysts, ≥0.04 inches/1 mm) in a finer grained matrix of glass (quenched melt) or sub-millimeter crystals called groundmass. Chemical analyses of “whole rock” geologic specimens, such as rocks or individual pumice lumps, typically are performed on finely pulverized samples. Whole-rock compositions yield information about magma source regions and tell whether new erupted ma-terial is the same or different from that previously erupted by the volcano. Often, composition will shift subtly during an eruption, heralding a new eruptive style or participation of melt from a different part of the magma system.
In addition to whole-rock chemical analysis, geologists use microphotographs and compositions of individual crystals and glass to further unravel rocks’ magmatic histories. High-resolution images of rock textures or chemical analyses of microscopic regions of crystals are obtained with tools broadly classified as microbeam instruments because the analyzed area is excited or extracted by an electron, ion, or light beam. For example, Fourier Transform Infrared Spectrometry (FTIR) can determine water and carbon dioxide concentrations in glass and minerals. Combining these concentrations with models of volatile (e.g., water and carbon dioxide) solubility in silicate melts allows estimates of pressure (and thus depth) of pre-eruptive magma storage. Mineral and glass compositions can also be used to estimate pressure and temperature conditions of crystallization, using geobarometers and geothermometers, respectively.
Finally, petrologists (geologists who specialize in the chemistry of rocks) conduct laboratory experiments that replicate pre-eruptive magma conditions (temperature, pressure, volatile contents, even decompression rates that mimic magma ascent). By varying experimental conditions in order to form minerals and melt found in natural rock samples, we can determine storage and ascent conditions for particular magma compositions and systems. Together, these types of studies give us both a time and space history of the movement of magma beneath a volcano towards the surface.
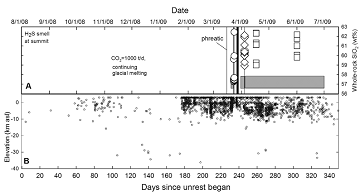
Figure 3. Silicon dioxide content of erupted andesite (A) and depths of located earthquakes (B) charted during the 2009 eruption of Redoubt. In (A), vertical lines are explosions, grey bars indicate periods of lava effusion, circles represent tephra from explosions, diamonds are samples from April 4 explosion, and squares are lavas from the final dome. Timing of hydrogen sulfide (H2S) smell and rates of carbon dioxide (CO2) are noted. Initial explosion on March 15 was gas-rich (phreatic); no new magma was ejected. Note that eruptive products become more SiO2-rich with time.
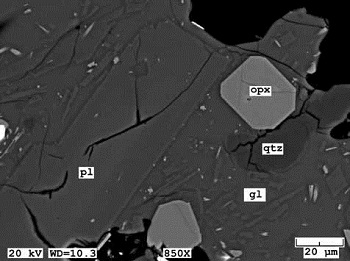
Case Studies
2009 eruption of Redoubt Volcano
Redoubt Volcano is an ice-covered, 10,197-ft (3,108-m) high stratovolcano 110 miles (177 km) southwest of Anchorage, Alaska, on the west side of Cook Inlet (Figure 2). Redoubt has erupted four times historically prior to 2009: in 1902, 1933, 1966-68, and 1989-90. The last two eruptions included a sequence of lava dome extrusions and multiple explosive events that produced ash plumes higher than 40,000 ft (12 km) above sea level (ASL). In addition, they produced floods and lahars that swept down the Drift River valley and impacted an oil storage facility at the coast 22 miles (35 km) distant.
The 2009 eruption was preceded by about eight months of low-level seismic activity, volcanic gas emissions, and steady melting of summit ice (Schaefer 2011). January 29, 2009, marked the onset of six weeks of volcanic tremor and shallow repeating earthquakes. Eighteen explosive events from March 22-28 produced ash columns between 17,000 and 62,000 ft (5.2 and 18.9 km) ASL, ash fall (Figure 1), and pyroclastic flows down the volcano’s north flank. Lahars, with flow depths to 33 ft (10 m) in the upper Drift River valley, inundated the Drift River oil terminal on March 23 and smaller lahars were observed on March 26, 27, and 28.
Dome growth starting on March 28 was followed by an explosion on April 4 that produced an ash cloud, pyroclastic flows on the volcano’s north flank, and voluminous lahars down the Drift River valley. Fine-grained, blocky ash that was erupted from this event suggests that it was triggered by collapse and depressurization of the new lava dome. Following the final explosive event, a final lava dome grew in the crater through July 1, 2009. The total magma volume erupted in 2009 is estimated to be about 0.02 cubic miles (0.1 km3) (Bull and Buurman In review).
The 2009 magmas are compositionally classified as andesites, typical for Redoubt and other subduction-zone volcanoes. They are rich in crystals such as plagioclase feldspar and range from 57.5 to 62.5 weight percentage (wt%) silicon oxide (SiO2) (Coombs et al. In review.) (Figure 3). Magmas from the early 2009 explosions and domes are almost all low-silica andesites (<58 wt% SiO2), but a transition to higher SiO2 and cooler magmas by about March 26 corresponded to a switch to predominantly effusive dome growth.
Comparison of plagioclase feldspar and melt (glass) compositions (Figure 4) from the andesites with results of experiments are consistent with pre-eruptive storage 2.5-3.7 miles (4-6 km) below the surface, coincident with many earthquakes located during the eruption (Figure 5). We interpret this as evidence that the 2009 low-silica andesite ascended from depth during the eight or more months prior to the first eruption, stalling and accumulating in the upper crust where its crystal rim and enclosing melt compositions were established. Ascent of this magma mobilized shallow, cooler, pre-existing magma pockets that fed the subsequently erupted lava domes. These latter magmas have compositions that suggest they are “leftovers” from previous eruptions. This pattern of newer melt “flushing” leftover magma from previous eruptions may be typical of small-volume dome-forming eruptions in Alaska and elsewhere.
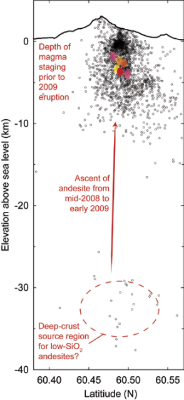
Figure 5. (Right) North-south cross section through Redoubt Volcano showing schematic pre-eruptive magma storage. Black circles represent hypocenters of earthquakes from mid-2008 through December 2009, as located by the Alaska Volcano Observatory. Scattered earthquakes between 19 and 25 miles (30-40 km) depth are “deep long-period” events that may represent withdrawal of magma from this depth as it began to rise towards the surface (Power et al. In review). The red oval between 1.2 and 2.5 miles (2-4 km) below sea level represents new low-silica andesite that arrived and erupted in 2009. Other colored ovals represent older, stagnant magma pods, some of which were remobilized and erupted in 2009 as well. All have been enlarged for clarity.
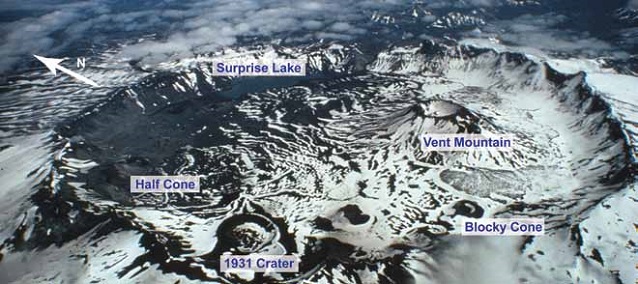
NPS photograph by M. Williams
Aniakchak 1931 eruption
Aniakchak caldera is a striking physical feature on the central Alaska Peninsula, 420 miles (670 km) southwest of Anchorage (Figure 2). The caldera formed by collapse of the Aniakchak volcano during a major eruption approxi-mately 3,400 years B.P. (before present) (Miller and Smith 1987). It was notable for its voluminous, composition-ally zoned pyroclastic-flow deposit of cream-colored rhyodacite overlain by dark gray andesite. Since then, postcaldera volcanism has formed four lava domes, three tuff cones, a small scoria cone, and the larger edifices of Half Cone and Vent Mountain within the 6-mile-diameter (10 km) caldera (Neal et al. 2001) (Figure 6).
The most recent eruption of Aniakchak took place during May and June 1931. The six-week-long eruption, the strongest from May 1-11, rained “egg-sized” rock fragments 19 miles (30 km) to the west in Port Heiden, left millimeters of ash as far as Kodiak Island, and repeatedly interfered with radio communications in the area. New lava flows were emplaced in the Main (1931) crater and as small flows at Doublet crater and Slag Heap toward the end of the activity. The total volume of erupted magma was about 0.07 cubic miles (0.3 km3) (Nicholson et al. 2011), less than 1% of which was lava flows. Similar to a circa 400 year B.P. eruption of Half Cone (Figure 7), the 1931 Aniakchak eruption began with relatively silica-rich dacitic magma (as much as 69 wt.% SiO2) and ended with relatively silica-poor basaltic andesitic magma (as little as 56 wt.% SiO2) (Nicholson et al. 2011).
Chemical analysis of crystals and their glass inclu-sions in Aniakchak postcaldera eruption products yield estimates of magma temperatures, dissolved volatile concentrations, and storage depths (Bacon 2002) (Figure 8). Results indicate that dacite magma resided at only 2 miles (3 km) depth prior to the circa 400 year B.P. explo-sive eruption of Half Cone. The subsequent eruption of Blocky Cone produced basaltic andesite that had partially crystallized at about 2 miles depth, possibly as a response to eruptions of Vent Mountain and Half Cone having expelled a relatively large volume of dacitic magma. The 1931 eruption drew dacitic magma from about 3 miles (5 km) depth. Interestingly, analysis of interferometric synthetic aperture radar (InSAR) images from 1992-2002 by Kwoun et al. (2006) indicates subsidence of the caldera center with a source modeled at about 2.5 miles (4 km) depth that the authors attributed to contraction caused by cooling or degassing of a shallow magma body.
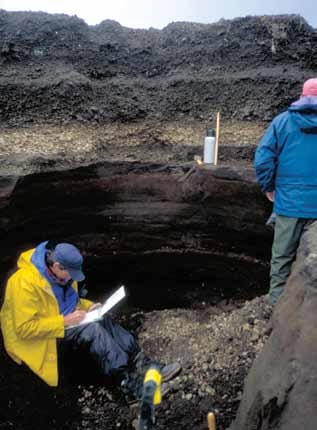
USGS photograph by C. Neal
Novarupta and the great eruption of 1912
The largest eruption of the twentieth century anywhere on Earth, the events of June 9-12, 1912, left an indelible mark on the landscape of the Katmai region. The eruption produced 3.4 cubic miles (14 km3) of magma, deposited more than a foot of ash on Kodiak, and lowered temperature in the northern hemisphere by as much as 1.8°F (1°C) for a year (Hildreth and Fierstein 2012). This eruption has intrigued geologists because of its size, great compositional diversity, and unusual magma plumbing system. Most notably, some magma was withdrawn from beneath Mount Katmai volcano, despite nearly all being erupted at Novarupta, 6 miles (10 km) away (Figure 9).
The eruption was preceded by several days of earthquakes felt in local villages, and on June 9, 2.2 cubic miles (9 km3) of high-silica rhyolite (77 wt% SiO2) erupted as pyroclastic flows and fall from a new vent, Novarupta. A few hours into the sequence, dacite and smaller amounts of andesite erupted, and Mount Katmai collapsed to form a 2-mile-wide caldera.
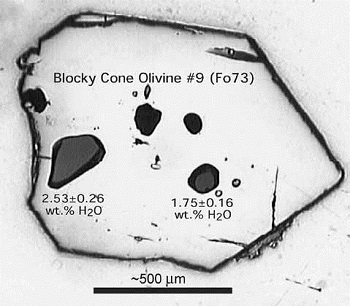
Recent studies have proposed contrasting models for the plumbing system that fed the 1912 eruption. Hildreth and Fierstein (2012) posit a single, compositionally zoned chamber that resided beneath Mount Katmai. Analyses of melt inclusions in crystals from the rhyolite suggest that the magma was fluid saturated (“gas” bubbles present) and contained ~4 wt% water dissolved in the melt. This is consistent with experiments on rhyolite pumice that show at those temperatures the rhyolite magma was stable between depths of 1.1-2.7 miles (1.8-4.4 km) below the surface (Coombs and Gardner 2001). Similar experiments on the 1912 andesite and dacite indicate that these magmas were also stored at a relatively shallow depth (Hammer et al. 2002), perhaps even more so than the rhyolite. These results are somewhat difficult to reconcile with a single zoned chamber, where denser andesite and dacite would reside below rhyolite. Deeper storage for the andesite and dacite was possible, however, if they contained appreciable carbon dioxide (CO2) in addition to water.
An alternate hypothesis is that multiple, separate magma bodies were tapped, though their pre-eruption locations are not clear. Geophysical evidence from the last several years suggests low-velocity zones consistent with magma beneath Katmai and near Trident, but not under Novarupta itself (Thurber et al., this issue). The configuration of the magma plumb-ing system for this unusual eruption is still not fully resolved and remains the topic of active research.
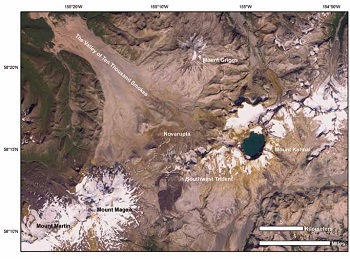
Image processed by Steve J. Smith
1953-1974 eruption of Trident Volcano
In winter 1953, a new vent formed on the southwest flank of Trident Volcano, just south of Novarupta on the Pacific side of Katmai Pass (Figures 9-10). Ash was ejected as high as 40,000 ft (12 km) ASL, and lava effused from the vent over the next several months. Activity at this new vent, coined Southwest Trident, continued in the form of ash eruptions and lava flow effusion intermittently through 1960, and sporadic explosions occurred for 14 years after, making the eruption the longest known in historical times in Alaska.
Study of Trident lavas and pumice shows that the eruption commenced with andesite, and that the magma became progressively more silica-rich through time (Coombs et al. 2000), similar to the 2009 eruption of Redoubt. Compositional profiles across olivine and mag-netite crystals within the lavas suggest that in the months prior to the 1953 eruption, a new andesite magma invaded a shallow dacitic magma reservoir. This process may have triggered the eruption by releasing heat and gas from the andesite into the dacite causing overpressure within the reservoir. Experiments and melt inclusions suggest that the mingled magmas were stored at a depth of approxi-mately 2.5 mi (4 km) below the surface prior to eruption.
Unlike the exceptional eruption of 1912, the modest (0.2 miles3/ 0.7 km3) eruption of Southwest Trident prob-ably represents a more typical Katmai-region eruption—in size, eruptive style, and magma composition. Scientists anticipate that the next eruption in this area could follow a similar pattern. And while modest in size, it is wise to remember that the eruption did continue for over two decades, and another such event could pose long-term hazards and nuisance in the region, especially to aviation.
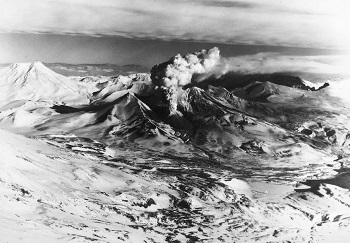
Photograph courtesy of the U.S. Navy
Conclusions
Volcanic rocks from recent eruptions tell a story of magma rising and “staging” in the upper levels of the earth’s crust prior to ultimately erupting or cooling in place. The residence time for such shallow magmas may range from thousands to hundreds of thousands of years in the largest caldera systems to as short as weeks or months in smaller reservoirs. In some cases, there is evidence that some magma was newly introduced into a shallow magma reservoir shortly prior to the eruption. The Alaska Volcano Observatory’s seismic networks and other monitoring techniques can often detect precursory signals associated with magma on the move. These signals can be used in tandem with insights gleaned from the rocks themselves to assist scientists in forecasting future eruptions and warning of the associated hazards.
For more information, visit the Alaska Volcano Observatory website.
References
Bacon, C.R. 2002. Depths of magma reservoirs inferred from preeruptive dissolved volatiles in the most recent postcaldera eruptions of Aniakchak volcano, Alaska. EOS, Transactions American Geophysical Union 83: S378.
Bull, K., and H. Buurman. In review. An overview of the 2009 eruption of Redoubt Volcano, Alaska. Journal of Volcanology and Geothermal Research.
Coombs, M.L., J.C. Eichelberger, and M.J. Rutherford. 2000. Magma storage and mixing conditions for the 1953-1974 eruptions of Southwest Trident volcano, Katmai National Park, Alaska. Contributions to Mineralogy and Petrology 140: 99-118.
Coombs, M.L., and J.E. Gardner. 2001. Shallow-storage conditions for the rhyolite of the 1912 eruption at Novarupta, Alaska. Geology 29: 775-778.
Coombs, M.L., T.W. Sisson, H. Bleick, S. Henton, C.J. Nye, A. Payne, C. Cameron, J.F. Larsen, K. Wallace, and K. Bull. In review. Andesites of the 2009 eruption of Redoubt Volcano, Alaska. Journal of Volcanology and Geothermal Research.
Hammer, J.E., M.J. Rutherford, and W. Hildreth. 2002. Magma storage prior to the 1912 eruption at Novarupta, Alaska. Contributions to Mineralogy and Petrology 144: 144-162.
Hildreth, W., and J. Fierstein. 2012. The Novarupta-Katmai eruption of 1912: Largest eruption of the twentieth century: Centennial perspectives. U.S. Geological Survey Professional Paper 1791.
Kwoun, O.-I., Z. Lu, C. Neal, and C. Wicks, Jr. 2006. Quiescent deformation of the Aniakchak caldera, Alaska. Geology 34: 5-8.
Neal, C., R.G. McGimsey, T.P. Miller, J.R. Riehle, and C.F. Waythomas. 2001. Preliminary volcano-hazard assessment for Aniakchak Volcano, Alaska. U.S. Geological Survey Open File Report 00-0519.
Nicholson, R.S., J.E. Gardner, and C.A. Neal. 2011. Variations in eruption style during the 1931 A.D. eruption of Aniakchak volcano, Alaska. Journal of Volcanology and Geothermal Research 207: 69-82.
Miller, T.P., and R.L. Smith. 1987. Late Quaternary caldera-forming eruptions in the eastern Aleutian arc, Alaska. Geology 15: 434-438.
Power, J.A., S.D. Stihler, B.A. Chouet, M.M. Haney, and D.M. Ketner. In review. Seismic observations of Redoubt Volcano, Alaska, 1989-2010 and a conceptual model of the Redoubt magmatic system. Journal of Volcanology and Geothermal Research.
Schaefer, J.R. (ed.) 2011. The 2009 eruption of Redoubt Volcano, Alaska. Alaska Division of Geological and Geophysical Surveys Report of Investigations 2011-5.
Part of a series of articles titled Alaska Park Science - Volume 11 Issue 1: Volcanoes of Katmai and the Alaska Peninsula.
Last updated: June 21, 2016