Last updated: February 21, 2024
Article
Harmful Algal Blooms (HABs) on the Horizon: Protecting Lakes in Acadia National Park from the Threat of Harmful Algal Blooms
Second Century Stewardship, Schoodic Institute at Acadia National Park
Rachel A. Fowler1 , Matthew J. Farragher1, William G. Gawley2, Jasmine E. Saros1
1School of Biology & Ecology and Climate Change Institute, University of Maine, Orono, ME U.S.A. 04469
2National Park Service, Acadia National Park, Bar Harbor, ME U.S.A. 04609
Building on these findings, we developed a scorecard to assess the individual risk of all ANP lakes to future HAB formation. To develop this risk assessment scorecard, we included parameters with known values for lakes in ANP that have been shown to affect vulnerability to HABs, such as trophic status, flushing rate, maximum depth, stratification, and level of protection within the ANP boundary. We weighted the parameters based on their relative importance in HAB development, then categorized the resulting ranks into risk levels of “low,” “moderate,” and “considerable.” Four of the 6 lakes that serve as drinking water supplies were in the low-risk category, while 2 drinking water lakes were at moderate risk. The risk assessment scorecard supports our findings that lakes like Jordan Pond have the fewest risk factors to future HAB development, while lakes like Witch Hole Pond have more risk factors and may be more vulnerable to developing HABs in the future. Based on the ranking of lakes on the Risk Assessment Scorecard, we suggest integrating sustainable, easily repeatable, and easy-to-implement steps into the current Air & Water monitoring program. We make recommendations about phycocyanin monitoring, winter sampling, and eDNA identification of cyanobacterial taxa, as well as the types of lakes in which to prioritize these data collection efforts. Educational outreach and citizen science will be important venues for public awareness and support. Together these efforts will help protect water quality and safeguard visitors and those who rely on ANP lakes as drinking water sources.
In recent years, several Maine lakes experienced unprecedented and unexpected cyanobacterial HABs (Wight 2019). Some of these lakes serve as drinking water sources, and required costly treatment plans to protect the public (Kidd 2019). National Parks in the Great Lakes region (Isle Royale, Pictured Rocks, and Sleeping Bear Dunes National Lakeshore) support ongoing research projects to study HABs and cyanotoxins in water resources (National Park Service (NPS) 2018). These parks receive approximately 1.6 million visitors annually, less than half of the 3.9 million visitors to Acadia National Park (ANP) in 2022 (Gibson 2023). In ANP, lakes are treasured for their aesthetic, recreational, educational, and scientific value, and six lakes in ANP watersheds are part of three municipal water systems. ANP lakes have excellent water quality, but when water quality issues develop, they typically occur quickly and across many lakes (Wight 2019).
Scientific consensus suggests that HABs are increasing worldwide (Heisler et al. 2008; Ho & Michalak 2017), and that with ongoing changes in climate, HABs will continue to become more frequent (Kudela et al. 2015; Michalak 2016; Carpenter & Pace 2018). Northern lakes (>45° N ANP is located at 44.3°N) are expected to undergo the greatest rates of change (Creed et al. 2018), with increasing temperatures and more frequent and severe precipitation events (Hanson et al. 2006; Kirtman et al. 2013). Lakes sequester natural and anthropogenic airshed and watershed nutrient inputs mediated by climate, hydrology, geography, and geology, and site-specific vulnerability to HABs is determined by physical, chemical, and biological lake characteristics (Paerl et al. 2018). Multiple internal and external factors can promote or suppress freshwater blooms, complicating predictions about HAB formation, especially in lakes with unknown bloom thresholds (Buelo et al. 2018).
Lake water temperature closely follows air temperature, and over the last century, air temperature across Maine has increased 3°F (Fernandez et al. 2015). This widespread increase in temperature might encourage bloom conditions for several reasons. Cyanobacteria can outcompete other algal groups in warm water (Weyhenmeyer 2001; Paerl & Huisman 2008), and warmer surface waters can increase thermal vertical stratification, which may favor toxic cyanobacteria taxa that are able to control buoyancy, like Dolichospermum and Microcystis (Gobler et al. 2007; Watson et al. 2015; Lofton et al. 2020). Warm water holds less dissolved oxygen than cold water, and lakes trending toward warmer water with lower dissolved oxygen may be at higher risk of HAB formation (U.S. EPA 2019a). Oxygen is consumed when cyanobacterial biomass decays at the conclusion of a bloom, which may lead to bottom-water anoxia (Paerl et al. 2001) and trigger release of sedimentary P in some lakes (Trimbee & Prepas 1988).
Shifts in winter temperature patterns affect the timing of ice-off and the length of the growing season (Wiedner et al. 2007; Deng et al. 2014; Watson et al. 2015). Maine lakes are sensitive to high winter and spring temperatures, and exhibit temporal synchrony of ice-off (Beyene & Jain 2015; Boeff et al. 2016), a pattern also documented in other boreal lakes (Magnuson et al. 2005). Recent ice-off in Maine lakes is generally occurring earlier (Hodgkins et al. 2002; Boeff et al. 2016), and is associated with the number of accumulated winter freezing and melting days (Beyene & Jain 2015). Warner et al. (2018) found that when ice-off occurred earlier in Jordan Pond in ANP, the period of spring turnover was longer, mixing depths were shallower, and the water column experienced strong thermal stratification, a trend observed in other boreal lakes (King et al. 1999). Stable thermal stratification favors buoyancy-regulating cyanobacterial taxa like Microcystis and Dolichospermum (Walsby et al. 1991) that can take advantage of light-rich surface waters and nutrient-rich bottom waters, and also facilitates surface accumulation of cyanobacterial cells (Paerl et al. 2001). Climate conditions that reduce snow cover on lake ice might reduce overwintering vegetative Microcystis cells (Brunberg & Blomqvist 2002) and increasing temperature could favor other cyanobacterial taxa over Microcystis, depending on light levels resulting from lake morphometry and/or water column stability (Carey et al. 2012a). Changes in wind speed affect thermal stratification and water column mixing, nutrient availability, and light levels (Zhang et al. 2021). Extreme precipitation events deliver pulses of dissolved organic carbon (DOC; Warner et al. 2020) and nutrients to surface waters, favoring cyanobacterial taxa that can store nutrients from these pulses, especially if the extreme precipitation events are followed by high temperatures and/or drought conditions (Jöhnk et al. 2008; Aubriot & Bonilla 2012; Huber et al. 2012; Watson et al. 2015).
Nutrient availability can also contribute to the development and strength of HABs, as cyanobacteria are strong competitors for phosphorus (P) and nitrogen (N; Paerl & Huisman 2008). Nutrients enter lakes through external sources like stormwater runoff, but can also be released through internal cycling from lake sediments when dissolved oxygen (DO) is depleted in bottom waters (Nürnberg 1984). The productivity, or trophic state, of a lake plays an important role in dissolved oxygen dynamics—productive lakes have higher nutrient levels and are more prone to anoxic bottom waters as summer progresses. Several multi-lake experiments have shown that cyanobacterial blooms are strongly associated with total phosphorus (TP), and that oligotrophic lakes with TP < 5 µg L-1 typically do not support HABs (Downing et al. 2001; Schindler 2012; Beaulieu et al. 2014; Watson et al. 2015). Other studies have suggested that the ratio of N:P is associated with toxin-producing HABs (Orihel et al. 2012; Harris et al. 2014; Watson et al. 2015). Cyanobacteria are the only phytoplankton group that can fix nitrogen—toxic, nitrogen-fixing taxa include Aphanizomenon, Dolichospermum, Cylindrospermopsis, Gloeotrichia, and Nodularia. Many cyanobacterial groups are also able to store luxury P, allowing them to thrive in both nutrient-limited and nutrient-rich conditions (Paerl et al. 2001). As Microcystis is unable to fix nitrogen, it is often associated with relatively high levels of N (Paerl et al. 2011; O’Neil et al. 2012).
However, cyanobacteria have a host of physiological adaptations across environmental conditions, and can also thrive in low-nutrient, oligotrophic lakes (Carey et al. 2012b; Watson et al. 2015; Reinl et al. 2021). Oligotrophic lakes may exhibit greater sensitivity to biogeochemical responses to anthropogenic climate change than higher-nutrient lakes (Reinl et al. 2021; Fowler et al. 2022), and there is much less known about the drivers of cyanobacterial blooms in oligotrophic lakes, especially northern lakes with cooler water temperature (Creed et al. 2018). Freshwater cyanobacteria have increased across a variety of lake morphometries in oligotrophic lakes in Sweden (Freeman et al. 2020) and Ontario (Winter et al. 2011; Sorichetti et al. 2014b), and a cyanobacterial bloom was documented in an oligo-mesotrophic lake in Germany (Uveges et al. 2012).
Data from the EPA National Lake Assessment show that the relative importance of nutrients and temperature on cyanobacterial biovolume varied by lake trophic state and cyanobacterial taxon (Rigosi et al. 2014). Nutrients were the most important driver of cyanobacterial biovolume in oligotrophic lakes, while temperature was a significant driver in mesotrophic lakes, and the interaction of nutrients and temperature were significant in eutrophic lakes. Microcystis was most responsive to temperature and Dolichospermum and Aphanizomenon were most sensitive to nutrient levels (Rigosi et al. 2014). Cyanobacterial blooms likely do not develop in response to a singular driver, but may form in response to high temperature at high nutrient levels, for example (Brookes & Carey 2011; Rigosi et al. 2014).
In low-nutrient lakes, cyanobacteria with buoyancy regulation are able to access the nutrient-rich hypolimnion and optimal light and temperature conditions throughout the water column (Reinl et al. 2021). In northern lakes, increasing dissolved organic matter (DOM) from the landscape will likely cause a community shift favoring cyanobacteria in low-nutrient lakes, as some cyanobacteria are able to access nutrients bound to DOM (Creed et al. 2018). Fowler et al. (2022) showed paleolimnological evidence of DOM as a nutrient subsidy for algal groups including colonial cyanobacteria in Seal Cove Pond in ANP.
Water clarity, sometimes estimated by water color or turbidity, can also contribute to bloom formation, as clearer water allows for greater light availability for photosynthetic processes (Carpenter & Pace 2018). Many cyanobacterial taxa use photo-protective pigments to survive high irradiance, and at the same time, to shade algal populations deeper in the water column (Paerl et al. 1983), and some taxa use photo-adaptive mechanisms to adjust to low-light conditions (Watson et al. 2015).
Historically, HABs have proven difficult to predict, identify, and manage (Watson et al. 2015). A multitude of physical, chemical, and biological variables contribute to population dynamics of blooming and non-blooming assemblages, which can co-occur and vary widely temporally and spatially (Watson et al. 2015). Strategies that incorporate multiple methods for quantifying and monitoring HABs are most effective (Elser et al. 1999; Lopez et al. 2008; Watson et al. 2015). Carpenter & Pace (2018) evaluated the potential for interpreting early warning signs of HABs in two small lakes in forested, undisturbed watersheds in Michigan. They showed that direct measurements of chlorophyll a (a measure of algal biomass), phycocyanin (a pigment produced by cyanobacteria), and dissolved oxygen saturation were reliable early warning indicators of blooms, and that these variables exhibited strong synchrony during blooms (Carpenter & Pace 2018).
Vulnerability assessments of resources’ sensitivity and adaptive capacity to climate change are an important piece in NPS management and planning strategies (Monahan & Fisichelli 2014), as resource vulnerability is a function of exposure, sensitivity, and adaptive capacity (IPCC 2007; Dawson et al. 2011; Gonzalez et al. 2018). While it is sometimes desirable to maximize precision of NPS resource risk assessments by incorporating many individual sites across a landscape, it may be most useful for policy and planning to maximize generality and realism at the cost of precision (Monahan & Fisichelli 2014). Therefore, our objective was to harness a multi-tool approach to create a risk assessment for a gradient of ANP lake types. Building upon the decades-long legacy of water quality monitoring in ANP, we used multiple monitoring and prediction tools to develop an early warning system to identify which lakes in ANP may be most susceptible to HAB formation and to understand how underlying environmental and lake conditions may trigger HAB development in vulnerable lakes.
The effect of warming on lakes might be regulated by lake trophic status and changes in nutrient loading (Arheimer et al. 2005; Elliot 2012; Anneville et al. 2015), and research studies across a variety of lake types and trophic states will help improve understanding of resilience of lakes to algal blooms (Ortiz et al. 2020; Reinl et al. 2021). Lakes in ANP fall along a gradient of trophic states and protection from localized anthropogenic nutrient inputs: Jordan Pond is oligotrophic and fully protected (within the boundaries of ANP), Seal Cove Pond is oligo-mesotrophic and partially protected, and Witch Hole Pond is meso-eutrophic, often exceeding EPA nutrient criteria (Gawley & Wiggin 2016), and fully protected. To realize risk assessment objectives, we instrumented these three lakes with sensors to record high-frequency temperature and dissolved oxygen data, produced bathymetric maps for volumetric modeling of dissolved oxygen, and measured phycocyanin, an indicator of cyanobacteria concentration, throughout the ice-free growing season in 2020. These data were paired with meteorological data from a weather station on the Jordan Pond House. The project findings will assist resource managers in identifying which types of lakes in ANP (and potentially other NPS units) require special monitoring, and can be used as a guide for allocating limited monitoring resources. These efforts to identify vulnerable water resources and prevent HABs will protect water quality and safeguard visitors and those who rely on ANP lakes as drinking water sources.
Rachel A. Fowler1 , Matthew J. Farragher1, William G. Gawley2, Jasmine E. Saros1
1School of Biology & Ecology and Climate Change Institute, University of Maine, Orono, ME U.S.A. 04469
2National Park Service, Acadia National Park, Bar Harbor, ME U.S.A. 04609
Abstract
Toxin-producing cyanobacteria can form HABs in lakes, leading to unfavorable impacts on drinking water, recreation, tourism, and ecosystem structure and function. Lakes in ANP have excellent water quality, but in the last decade several Maine lakes have experienced unprecedented and costly HABs, as have lakes in other National Park Service (NPS) units. To assess early warning signs in lakes varying in trophic status and other water quality parameters, we sampled Jordan Pond, Seal Cove Pond, and Witch Hole Pond in 2020. We 1) instrumented each lake with temperature and dissolved oxygen sensors for high-resolution data; 2) performed biweekly water column sampling; 3) tracked phycocyanin (an indicator of cyanobacteria) concentration; 4) prepared bathymetric maps of each lake; 5) collected meteorological data; and 6) assessed long-term monitoring data collected by the ANP Air & Water Quality Program. We prepared an assessment of each lake type that evaluated risk conditions including temperature, dissolved oxygen, chlorophyll a (chl a) and phycocyanin concentration, and nutrient levels. We also considered how the effects of lake morphometry could affect susceptibility to HABs. Shallow, eutrophic lakes like Witch Hole Pond could be more vulnerable to HAB formation due to high surface and water column temperature, low dissolved oxygen, high nutrients, and greater relative concentrations of chl a and phycocyanin. Jordan Pond, a deep oligotrophic lake, did not exhibit early warning signs of HABs, and we expect that similar lakes in ANP are equally resistant to HAB conditions.Building on these findings, we developed a scorecard to assess the individual risk of all ANP lakes to future HAB formation. To develop this risk assessment scorecard, we included parameters with known values for lakes in ANP that have been shown to affect vulnerability to HABs, such as trophic status, flushing rate, maximum depth, stratification, and level of protection within the ANP boundary. We weighted the parameters based on their relative importance in HAB development, then categorized the resulting ranks into risk levels of “low,” “moderate,” and “considerable.” Four of the 6 lakes that serve as drinking water supplies were in the low-risk category, while 2 drinking water lakes were at moderate risk. The risk assessment scorecard supports our findings that lakes like Jordan Pond have the fewest risk factors to future HAB development, while lakes like Witch Hole Pond have more risk factors and may be more vulnerable to developing HABs in the future. Based on the ranking of lakes on the Risk Assessment Scorecard, we suggest integrating sustainable, easily repeatable, and easy-to-implement steps into the current Air & Water monitoring program. We make recommendations about phycocyanin monitoring, winter sampling, and eDNA identification of cyanobacterial taxa, as well as the types of lakes in which to prioritize these data collection efforts. Educational outreach and citizen science will be important venues for public awareness and support. Together these efforts will help protect water quality and safeguard visitors and those who rely on ANP lakes as drinking water sources.
Introduction
Globally, harmful algal blooms (HABs) are increasing rapidly in inland waters (Watson et al. 2015). HABs cause unfavorable and costly impacts to drinking water resources, tourism and recreation, property values, and ecosystem structure and function (Watson et al. 2015). Lake scientists and resource managers in federal and state agencies in Maine are concerned about the vulnerability of lakes to cyanobacterial HABs, which develop when cyanobacteria are present in lake water and produce toxins. The toxins may reach sufficient concentrations to harm humans, livestock, and pets who come into contact with the water. Some common toxin-producing, bloom-forming cyanobacteria occurring in Maine lakes are Dolichospermum (formerly Anabaena), Aphanizomenon, Gloeotrichia, Microcystis, and Oscillatoria (Carey et al. 2012b; Bacon 2016). These taxa can produce hepatotoxins and neurotoxins, and can cause irritation of mucous membranes in exposed individuals (Watson et al. 2015). Algal blooms can also cause taste and odor issues in drinking water sources. The WHO, CDC, and EPA describe HABs as an emerging public health issue and major environmental concern (Chorus & Welker 2021; CDC 2023; U.S. EPA 2023b). In Maine and the rest of the northeast U.S., microcystin-LR is one of the most common and harmful toxins occurring in cyanobacterial HABs, and concentrations of microcystin-LR exceeded EPA and WHO drinking water standards in a subset of Maine lakes in 2009 (Bacon 2016).In recent years, several Maine lakes experienced unprecedented and unexpected cyanobacterial HABs (Wight 2019). Some of these lakes serve as drinking water sources, and required costly treatment plans to protect the public (Kidd 2019). National Parks in the Great Lakes region (Isle Royale, Pictured Rocks, and Sleeping Bear Dunes National Lakeshore) support ongoing research projects to study HABs and cyanotoxins in water resources (National Park Service (NPS) 2018). These parks receive approximately 1.6 million visitors annually, less than half of the 3.9 million visitors to Acadia National Park (ANP) in 2022 (Gibson 2023). In ANP, lakes are treasured for their aesthetic, recreational, educational, and scientific value, and six lakes in ANP watersheds are part of three municipal water systems. ANP lakes have excellent water quality, but when water quality issues develop, they typically occur quickly and across many lakes (Wight 2019).
Scientific consensus suggests that HABs are increasing worldwide (Heisler et al. 2008; Ho & Michalak 2017), and that with ongoing changes in climate, HABs will continue to become more frequent (Kudela et al. 2015; Michalak 2016; Carpenter & Pace 2018). Northern lakes (>45° N ANP is located at 44.3°N) are expected to undergo the greatest rates of change (Creed et al. 2018), with increasing temperatures and more frequent and severe precipitation events (Hanson et al. 2006; Kirtman et al. 2013). Lakes sequester natural and anthropogenic airshed and watershed nutrient inputs mediated by climate, hydrology, geography, and geology, and site-specific vulnerability to HABs is determined by physical, chemical, and biological lake characteristics (Paerl et al. 2018). Multiple internal and external factors can promote or suppress freshwater blooms, complicating predictions about HAB formation, especially in lakes with unknown bloom thresholds (Buelo et al. 2018).
Lake water temperature closely follows air temperature, and over the last century, air temperature across Maine has increased 3°F (Fernandez et al. 2015). This widespread increase in temperature might encourage bloom conditions for several reasons. Cyanobacteria can outcompete other algal groups in warm water (Weyhenmeyer 2001; Paerl & Huisman 2008), and warmer surface waters can increase thermal vertical stratification, which may favor toxic cyanobacteria taxa that are able to control buoyancy, like Dolichospermum and Microcystis (Gobler et al. 2007; Watson et al. 2015; Lofton et al. 2020). Warm water holds less dissolved oxygen than cold water, and lakes trending toward warmer water with lower dissolved oxygen may be at higher risk of HAB formation (U.S. EPA 2019a). Oxygen is consumed when cyanobacterial biomass decays at the conclusion of a bloom, which may lead to bottom-water anoxia (Paerl et al. 2001) and trigger release of sedimentary P in some lakes (Trimbee & Prepas 1988).
Shifts in winter temperature patterns affect the timing of ice-off and the length of the growing season (Wiedner et al. 2007; Deng et al. 2014; Watson et al. 2015). Maine lakes are sensitive to high winter and spring temperatures, and exhibit temporal synchrony of ice-off (Beyene & Jain 2015; Boeff et al. 2016), a pattern also documented in other boreal lakes (Magnuson et al. 2005). Recent ice-off in Maine lakes is generally occurring earlier (Hodgkins et al. 2002; Boeff et al. 2016), and is associated with the number of accumulated winter freezing and melting days (Beyene & Jain 2015). Warner et al. (2018) found that when ice-off occurred earlier in Jordan Pond in ANP, the period of spring turnover was longer, mixing depths were shallower, and the water column experienced strong thermal stratification, a trend observed in other boreal lakes (King et al. 1999). Stable thermal stratification favors buoyancy-regulating cyanobacterial taxa like Microcystis and Dolichospermum (Walsby et al. 1991) that can take advantage of light-rich surface waters and nutrient-rich bottom waters, and also facilitates surface accumulation of cyanobacterial cells (Paerl et al. 2001). Climate conditions that reduce snow cover on lake ice might reduce overwintering vegetative Microcystis cells (Brunberg & Blomqvist 2002) and increasing temperature could favor other cyanobacterial taxa over Microcystis, depending on light levels resulting from lake morphometry and/or water column stability (Carey et al. 2012a). Changes in wind speed affect thermal stratification and water column mixing, nutrient availability, and light levels (Zhang et al. 2021). Extreme precipitation events deliver pulses of dissolved organic carbon (DOC; Warner et al. 2020) and nutrients to surface waters, favoring cyanobacterial taxa that can store nutrients from these pulses, especially if the extreme precipitation events are followed by high temperatures and/or drought conditions (Jöhnk et al. 2008; Aubriot & Bonilla 2012; Huber et al. 2012; Watson et al. 2015).
Nutrient availability can also contribute to the development and strength of HABs, as cyanobacteria are strong competitors for phosphorus (P) and nitrogen (N; Paerl & Huisman 2008). Nutrients enter lakes through external sources like stormwater runoff, but can also be released through internal cycling from lake sediments when dissolved oxygen (DO) is depleted in bottom waters (Nürnberg 1984). The productivity, or trophic state, of a lake plays an important role in dissolved oxygen dynamics—productive lakes have higher nutrient levels and are more prone to anoxic bottom waters as summer progresses. Several multi-lake experiments have shown that cyanobacterial blooms are strongly associated with total phosphorus (TP), and that oligotrophic lakes with TP < 5 µg L-1 typically do not support HABs (Downing et al. 2001; Schindler 2012; Beaulieu et al. 2014; Watson et al. 2015). Other studies have suggested that the ratio of N:P is associated with toxin-producing HABs (Orihel et al. 2012; Harris et al. 2014; Watson et al. 2015). Cyanobacteria are the only phytoplankton group that can fix nitrogen—toxic, nitrogen-fixing taxa include Aphanizomenon, Dolichospermum, Cylindrospermopsis, Gloeotrichia, and Nodularia. Many cyanobacterial groups are also able to store luxury P, allowing them to thrive in both nutrient-limited and nutrient-rich conditions (Paerl et al. 2001). As Microcystis is unable to fix nitrogen, it is often associated with relatively high levels of N (Paerl et al. 2011; O’Neil et al. 2012).
However, cyanobacteria have a host of physiological adaptations across environmental conditions, and can also thrive in low-nutrient, oligotrophic lakes (Carey et al. 2012b; Watson et al. 2015; Reinl et al. 2021). Oligotrophic lakes may exhibit greater sensitivity to biogeochemical responses to anthropogenic climate change than higher-nutrient lakes (Reinl et al. 2021; Fowler et al. 2022), and there is much less known about the drivers of cyanobacterial blooms in oligotrophic lakes, especially northern lakes with cooler water temperature (Creed et al. 2018). Freshwater cyanobacteria have increased across a variety of lake morphometries in oligotrophic lakes in Sweden (Freeman et al. 2020) and Ontario (Winter et al. 2011; Sorichetti et al. 2014b), and a cyanobacterial bloom was documented in an oligo-mesotrophic lake in Germany (Uveges et al. 2012).
Data from the EPA National Lake Assessment show that the relative importance of nutrients and temperature on cyanobacterial biovolume varied by lake trophic state and cyanobacterial taxon (Rigosi et al. 2014). Nutrients were the most important driver of cyanobacterial biovolume in oligotrophic lakes, while temperature was a significant driver in mesotrophic lakes, and the interaction of nutrients and temperature were significant in eutrophic lakes. Microcystis was most responsive to temperature and Dolichospermum and Aphanizomenon were most sensitive to nutrient levels (Rigosi et al. 2014). Cyanobacterial blooms likely do not develop in response to a singular driver, but may form in response to high temperature at high nutrient levels, for example (Brookes & Carey 2011; Rigosi et al. 2014).
In low-nutrient lakes, cyanobacteria with buoyancy regulation are able to access the nutrient-rich hypolimnion and optimal light and temperature conditions throughout the water column (Reinl et al. 2021). In northern lakes, increasing dissolved organic matter (DOM) from the landscape will likely cause a community shift favoring cyanobacteria in low-nutrient lakes, as some cyanobacteria are able to access nutrients bound to DOM (Creed et al. 2018). Fowler et al. (2022) showed paleolimnological evidence of DOM as a nutrient subsidy for algal groups including colonial cyanobacteria in Seal Cove Pond in ANP.
Water clarity, sometimes estimated by water color or turbidity, can also contribute to bloom formation, as clearer water allows for greater light availability for photosynthetic processes (Carpenter & Pace 2018). Many cyanobacterial taxa use photo-protective pigments to survive high irradiance, and at the same time, to shade algal populations deeper in the water column (Paerl et al. 1983), and some taxa use photo-adaptive mechanisms to adjust to low-light conditions (Watson et al. 2015).
Historically, HABs have proven difficult to predict, identify, and manage (Watson et al. 2015). A multitude of physical, chemical, and biological variables contribute to population dynamics of blooming and non-blooming assemblages, which can co-occur and vary widely temporally and spatially (Watson et al. 2015). Strategies that incorporate multiple methods for quantifying and monitoring HABs are most effective (Elser et al. 1999; Lopez et al. 2008; Watson et al. 2015). Carpenter & Pace (2018) evaluated the potential for interpreting early warning signs of HABs in two small lakes in forested, undisturbed watersheds in Michigan. They showed that direct measurements of chlorophyll a (a measure of algal biomass), phycocyanin (a pigment produced by cyanobacteria), and dissolved oxygen saturation were reliable early warning indicators of blooms, and that these variables exhibited strong synchrony during blooms (Carpenter & Pace 2018).
Vulnerability assessments of resources’ sensitivity and adaptive capacity to climate change are an important piece in NPS management and planning strategies (Monahan & Fisichelli 2014), as resource vulnerability is a function of exposure, sensitivity, and adaptive capacity (IPCC 2007; Dawson et al. 2011; Gonzalez et al. 2018). While it is sometimes desirable to maximize precision of NPS resource risk assessments by incorporating many individual sites across a landscape, it may be most useful for policy and planning to maximize generality and realism at the cost of precision (Monahan & Fisichelli 2014). Therefore, our objective was to harness a multi-tool approach to create a risk assessment for a gradient of ANP lake types. Building upon the decades-long legacy of water quality monitoring in ANP, we used multiple monitoring and prediction tools to develop an early warning system to identify which lakes in ANP may be most susceptible to HAB formation and to understand how underlying environmental and lake conditions may trigger HAB development in vulnerable lakes.
The effect of warming on lakes might be regulated by lake trophic status and changes in nutrient loading (Arheimer et al. 2005; Elliot 2012; Anneville et al. 2015), and research studies across a variety of lake types and trophic states will help improve understanding of resilience of lakes to algal blooms (Ortiz et al. 2020; Reinl et al. 2021). Lakes in ANP fall along a gradient of trophic states and protection from localized anthropogenic nutrient inputs: Jordan Pond is oligotrophic and fully protected (within the boundaries of ANP), Seal Cove Pond is oligo-mesotrophic and partially protected, and Witch Hole Pond is meso-eutrophic, often exceeding EPA nutrient criteria (Gawley & Wiggin 2016), and fully protected. To realize risk assessment objectives, we instrumented these three lakes with sensors to record high-frequency temperature and dissolved oxygen data, produced bathymetric maps for volumetric modeling of dissolved oxygen, and measured phycocyanin, an indicator of cyanobacteria concentration, throughout the ice-free growing season in 2020. These data were paired with meteorological data from a weather station on the Jordan Pond House. The project findings will assist resource managers in identifying which types of lakes in ANP (and potentially other NPS units) require special monitoring, and can be used as a guide for allocating limited monitoring resources. These efforts to identify vulnerable water resources and prevent HABs will protect water quality and safeguard visitors and those who rely on ANP lakes as drinking water sources.
Methods
Site Description
Three lakes in Acadia National Park were selected for this study based on trophic status and morphological features (Figure 1). Jordan Pond, an oligotrophic lake and drinking water source, is 76 ha with a mean depth of 21 m. Seal Cove Pond (105 ha in area; 4 m mean depth) is oligo-mesotrophic and Witch Hole Pond (10 ha in area; 2 m mean depth) is meso-eutrophic (Table 1). Jordan Pond and Witch Hole Pond are entirely within ANP boundaries and are protected from watershed development and other water and land use effects, while Seal Cove Pond has residential homes on its western shore.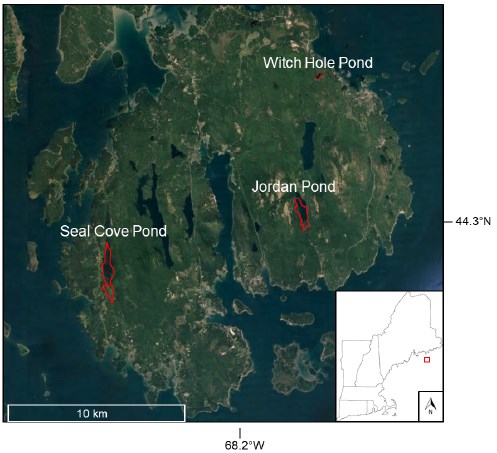
Site | Area (ha) | Mean / Maximum depth (m) | Chl a (ug L -1 ) | Secchi (m) | TP (ug L -1 ) | DOC (mg L -1 ) |
---|---|---|---|---|---|---|
Jordan Pond | 75.3 | 25.6 / 45.7 | 0.9 ± 0.3 | 15.1 ± 0.7 | 2.7 ± 1.1 | 1.8 ± 0.2 |
Seal Cove Pond | 103.2 | 5.5 / 13.4 | 2.3 ± 1.2 | 6.6 ± 1.4 | 4.7 ± 1.4 | 4.0 ± 0.4 |
Witch Hole Pond | 9.7 | 3.7 / 9.4 | 4.0 ± 1.8 | 4.9 ± 0.4 | 9.2 ± 3.0 | 3.6 ± 0.4 |
High-Frequency Sensors
Each lake was outfitted with a buoy equipped with high-frequency sensors for continuous monitoring throughout the season. The buoys were deployed in February 2020 through the iceand remained submerged through fall turnover in November 2020. Buoys were deployed suchthat one PME miniPAR® Logger was positioned 2 m below the water surface, one PMEminiDOT® Logger at 3 m below the water surface, and another PME miniDOT® Logger at 2 mabove the lake bottom. HOBO Pendant® MX Water Temperature Data Loggers were placed atevery 1-m interval. All PAR sensors, dissolved oxygen sensors, and temperature sensors weresynchronized to record data in 1-hour intervals. Buoys were deployed near the site of maximumdepth in Seal Cove Pond and Witch Hole Pond (13 m and 9 m, respectively) and at 32 m inJordan Pond. Meteorological data were collected from the Jordan Pond House weather station.Manual Sampling
Manual sampling was done once through the ice in February 2020, and was performed approximately bi-weekly from mid-May until November 2020. A YSI EXO3 Multi-Parameter Water Quality Sonde was used for profiles of temperature and dissolved oxygen at 1-m intervals. Continuous profiles of temperature, chlorophyll a fluorescence (fChl), phycocyanin fluorescence (fPhy), and colored dissolved organic matter (CDOM) were measured using a Turner Designs C3™ Submersible Fluorometer. Water transparency was measured using a Secchi disk. In addition, water samples were taken at three depths corresponding to the epi-, meta-, and hypolimnion for each profile to measure extracted chlorophyll biomass, phytoplankton identification and enumeration, and nutrients, including TP, NO3-, NH4+, and DOC. Water samples were filtered through 0.7-μm Whatman GF/F filters to determine concentrations of inorganic nutrients (NH4+, NO3-) and DOC. NH4+, NO3-, and DOC concentrations were determined using standard phenate, cadmium reduction, and catalytic oxidation methods, respectively (American Public Health Association 2000). Chlorophyll a concentration (chl a), a proxy for algal biomass, was measured by filtering whole water samples (kept in light-blocking bottles) through 0.7-μm Whatman GF/F filters, after which the filters were frozen for more than 24 hours, placed in 90% acetone, and analyzed using a UV-Visible spectrophotometer within two weeks of collection using standardized methods (American Public Health Association 2000). All water sample analyses were conducted at the University of Maine Sawyer Water Research Laboratory.Phytoplankton Cell Density
Phytoplankton identification and enumeration was performed for samples at three depths on four separate dates for both Jordan Pond and Seal Cove Pond. Subsamples of whole water samples were fixed in Lugol’s Iodine solution and stored in the dark until 20 to 25 ml was settled in an Utermöhl chamber for at least 12 hours. Phytoplankton were then counted along two transects at 600x magnification using a Nikon TS100 inverted microscope. Raw cell counts were converted to cell density (ml-1) based on subsample volume settled and the number of transects that were counted (Hillebrand et al. 1999). Individuals were identified to genus using Wehr et al. (2015), except for unidentified non-flagellated cells and unidentified cysts.Bathymetry
Bathymetric maps of the three study lakes were generated using ReefMaster® software using a Humminbird 597ci sonar device.Long-Term Data
Long-term datasets (1995-2020) for Secchi disk depth, dissolved oxygen, temperature, chlorophyll, and nutrients were obtained from the ANP NPS water quality monitoring program to evaluate risk assessments. Weather data (2013-2022) including wind speed, precipitation, and air temperature records from the Jordan Pond House weather station were used for historic comparisons of meteorological variables.Data Analysis
Data analyses were performed using R version 4.1.0 (R Core Team 2021). The function thermocline.depth was used from the package rLakeAnalyzer (Winslow et al. 2019).To create the risk assessment scorecard, we used ANP lake data compiled from the Maine Department of Environmental Protection and Maine Department of Inland Fisheries and Wildlife. A weighted average was created using the following variables: trophic status, flushing rate, maximum depth, whether the lake stratifies, and whether the lake is fully or partially protected within the ANP boundary. These parameters were categorized and weighted as follows:
Trophic state:
Oligotrophic = 1, Mesotrophic = 2, Eutrophic = 3Flushing rate:
>10 yr -1 = 1, 1 to 10 yr -1 = 2, < 1 yr -1 = 3
Maximum depth:
>25 m = 1, 10 to 25 m = 2, < 10 m = 3
Stratifies?
No = 1, Yes = 2
Fully protected within ANP boundary?
Yes = 1, No = 1.5
The weighted average produced unit-less values for each lake between 1.3 and 2.5. The lakes were sorted into risk assessment categories based on the following criteria:
Low concern: <1.8
Moderate concern: 1.8 to 2.0
Considerable concern >2.0
Results
Light
Average Secchi depth during the stratified period was 15.1 m in Jordan Pond (n=5), 6.6 m in Seal Cove Pond (n=5), and 4.9 m in Witch Hole Pond (n=5; Figure 2).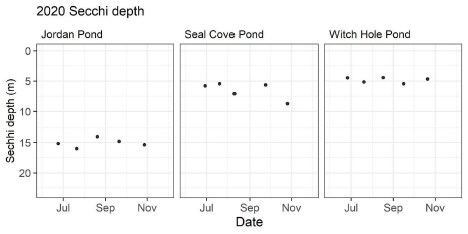
High-Frequency Sensor Summary Plots
Temperature, DO, phycocyanin fluorescence, and chlorophyll a fluorescence data from 1-m i nterval sensor water column profiles are summarized for Jordan Pond (Figure 3), Seal Cove Pond (Figure 4), and Witch Hole Pond (Figure 5).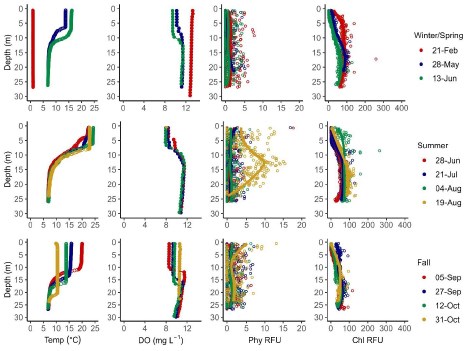
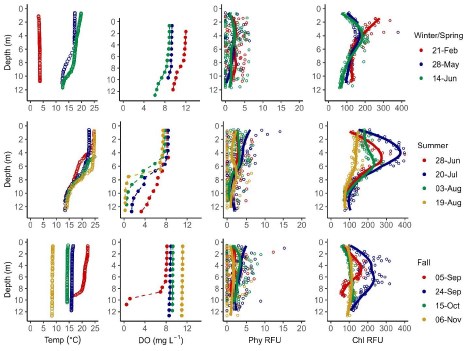
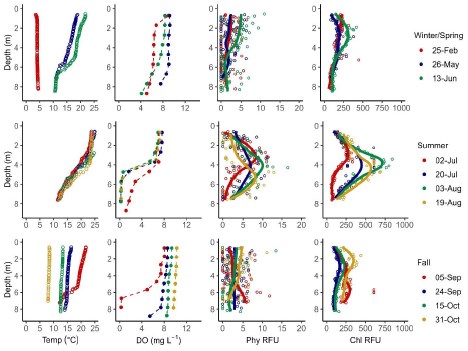
Temperature
In 2020, Jordan Pond, Seal Cove Pond and Witch Hole Pond thermally stratified on 5/28, 5/22, and 5/18, respectively (Figures 6, 7). We define the onset of stratification as the first day there is a ≥ 1°C difference per meter in the water column, as in Warner et al. (2018). In Jordan Pond, the maximum temperature of the top sensor was 24.9°C on 8/14/20. Maximum temperatures were 28.1°C on 7/31/20 in Seal Cove Pond and 28.6°C on 8/1/20 in Witch Hole Pond. Epilimnion temperatures were >25°C in Seal Cove Pond from 7/22/20 to 8/17/20 and in Witch Hole Pond from 7/19/20 to 8/17/20. After stratification, the temperature recorded by the bottom sensor remained at approximately 6.6°C for the remainder of the season in Jordan Pond. Temperature recorded by the bottom sensor increased throughout the summer to approximately 20°C and 16°C in Seal Cove Pond and Witch Hole Pond, respectively (Figure 6).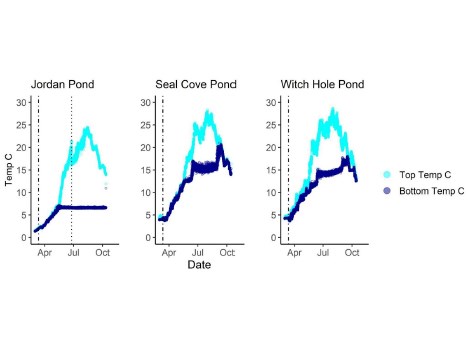
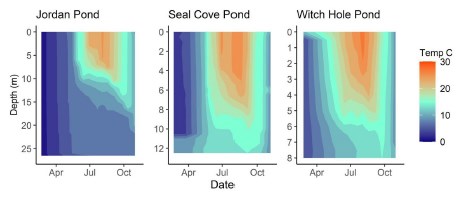
Dissolved Oxygen
Jordan Pond exhibited very different dissolved oxygen dynamics than Seal Cove Pond and Witch Hole Pond (Figures 8, 9). Both top and bottom sensors recorded 13.2 mg L - 1 dissolved oxygen (DO) in Jordan Pond at the beginning of March 2020, around the timing of ice out (Figure 8). Bottom-water DO gradually declined throughout the season, reaching 8.7 mg L - 1 at the beginning of October. Top-water DO gradually declined at the same rate as bottom-water DO until mid-May, when top-water DO decreased more steeply. The minimum DO recorded by the top sensor in Jordan Pond was 7.3 mg L - 1 at the end of August. During September, top-water DO in Jordan Pond increased to 10.8 mg L - 1. Seasonal DO patterns were different in Seal Cove Pond and Witch Hole Pond. After ice-out in Seal Cove Pond, both top and bottom sensors recorded 11 to 12 mg L - 1 DO, which decreased at the same rate for both sensors until the end of May, when the bottom sensor measured steeper rates of DO decline, until anoxia (<2mg L - 1 ) occurred during the last week of July and lasted until the beginning of September. During this time, DO at the top sensor remained relatively steady, averaging 7.7 mg L - 1 . At the beginning of September, bottom-water DO increased abruptly and matched top-water DO, 8.6 mg L - 1 . Patterns of top- and bottom-water DO in Witch Hole Pond were similar to those in Seal Cove Pond, but anoxia at the bottom DO sensor occurred earlier in the season, at the beginning of July, and lasted longer, through late September. DO measurements were more variable at the top sensor in Witch Hole Pond from July through September, averaging 7.5 mg L - 1.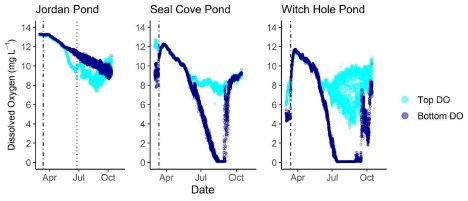
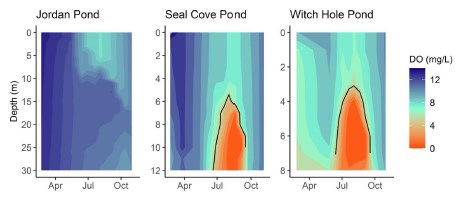
Phycocyanin
Water column measurements of phycocyanin fluorescence and interpolated values were low (<5 RFU) for most of the ice-free season in both Jordan Pond and Seal Cove Pond (Figure 10). However, the surface water of both lakes had peaks in phycocyanin fluorescence after ice-out, peaking at 104 RFU in Jordan Pond on 5/13/20 and 112 RFU in Seal Cove Pond on 3/25/20. Water column measurements were not taken from Witch Hole Pond on either of these dates, or from Jordan Pond on 3/25/20. Phycocyanin RFU peaked again at the surface of Jordan Pond on 7/21/20, reaching 172 RFU. Phycocyanin RFU peaked at 126 RFU at the surface of Seal Cove Pond on 9/5/20. Average water column (to 21 m) phycocyanin in Jordan Pond on 8/19/20 was 6 RFU. Maximum phycocyanin was 15 RFU at 13 m depth on that day. Water column phycocyanin was very low the rest of the ice-free season. In Seal Cove Pond, phycocyanin RFU was 7 RFU at 4 m depth on 7/20/20 and ranged from 0 to 7 RFU in the water column to 4 m depth on 9/24/20. In Witch Hole Pond, phycocyanin values were not as high in surface waters as in Jordan Pond and Seal Cove Pond. Phycocyanin RFU was generally higher throughout the water column in Witch Hole Pond than in Jordan Pond and Seal Cove Pond, especially during July and August. During this time, phycocyanin RFU increased to 13 RFU from 2 m to 6 m depth.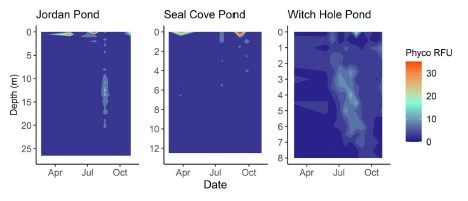
Chlorophyll a
Chlorophyll fluorescence trends were slightly different than phycocyanin fluorescence trends (Figure 11). In Jordan Pond, chlorophyll RFU was consistently low (<100 RFU) throughout the season except for one reading of 263 RFU on 8/4/20. At the surface of both Jordan Pond and Seal Cove Pond, chlorophyll fluorescence remained low throughout the season. Seal Cove Pond had a high chlorophyll fluorescence reading (600 RFU) immediately after ice-off on 3/25/20 at 8 m depth that represents a bloom of diatoms, and another peak on 7/20/20 at 4 m depth (510 RFU). Witch Hole Pond had the largest chlorophyll peak on 8/3/20 with 842 RFU at 3 m depth. Summer chlorophyll fluorescence in Witch Hole Pond was generally higher throughout the water column than in Jordan Pond and Seal Cove Pond.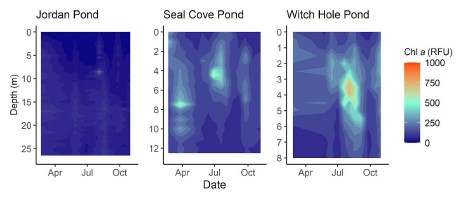
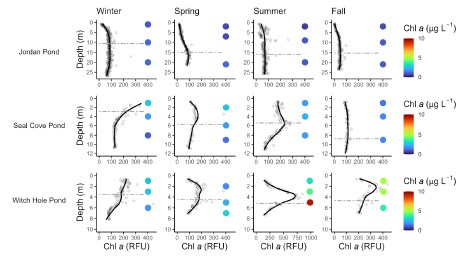
Nutrients & Dissolved Organic Carbon (DOC)
During the period of stratification, nutrients in Jordan Pond were generally low in the epi-, meta- and hypolimnion (Figure 13). Average epilimnion TP and TN were 2.7 µg L - 1 and 96 µg L - 1 , respectively (n=9). The ratio of dissolved inorganic nitrogen (DIN) to TP, which can be useful for l imiting nutrient limitation (Bergstrom 2010), was >3.4 throughout the water column for much of the sampling period (average=5.5), indicating phosphorus (P) limitation. In Seal Cove Pond, average epilimnion TP and TN were 4.7 µg L - 1 and 200 µg L - 1 , respectively (n=9). Most of the DIN:TP values for Seal Cove Pond fell between 1.5 to 3.4, with some higher values in the fall i ndicating possible P-limitation (average=2.1). In Witch Hole Pond, average epilimnion TP and TN were 9.2 µg L - 1 and 259 µg L - 1 , respectively (n=8). TP and NH 4 + concentrations in Witch Hole Pond were higher throughout all seasons at all depths than in Jordan Pond and Seal Cove Pond, and NO 3 - was similar to Seal Cove Pond and lower than in Jordan Pond in the summer and fall. The DIN:TP ratio of Witch Hole Pond indicates potential N-limitation in the epilimnion in the spring, at all depths in the summer, and in the epilimnion in the fall. Average epilimnion DIN:TP was 1.4.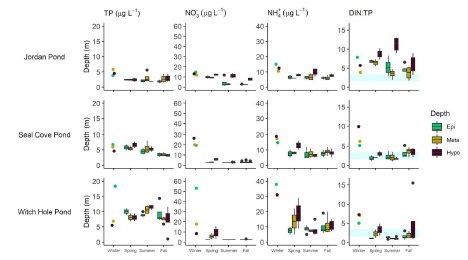
Phytoplankton Cell Density
Phytoplankton cell density was generally low (≤1000 cells ml - 1 ) during winter and spring sampling in Jordan Pond, with greater cell density in the hypolimnion (Figure 14). Cell density was high (~2000 cells ml - 1 ) in the epi- and metalimnia of Jordan Pond during the summer. During this time, there was a relatively high proportion of haptophytes, followed by diatoms. Approximately 8% of the phytoplankton cell density in the metalimnion during the summer was attributed to cyanobacteria, particularly Aphanocapsa and Aphanothece (Figure 15). These genera belong to different taxonomic orders than Aphanizomenon, but may still produce toxins under certain conditions. Phytoplankton cell density in Seal Cove Pond was highest in the epilimnion in the spring (~2400 cells ml - 1 ), and was primarily composed of chrysophytes, followed by cryptophytes. In the summer, cell density decreased to approximately 1100 cells ml - 1 i n the epilimnion of Seal Cove Pond, did not change in the metalimnion, and increased in the hypolimnion. The relative proportions of taxa changed at all depths. Haptophytes, dinoflagellates, and chrysophytes made up the greatest proportions of phytoplankton in the epi- and metalimnia, while haptophytes dominated in the hypolimnion. The relative proportion of cyanobacteria in Seal Cove Pond was low across depths and seasons. The greatest proportion of cyanobacteria in Seal Cove Pond was Dolichospermum i n the epilimnion during spring (~1% of phytoplankton cell density). We do not have phytoplankton cell density counts for Witch Hole Pond at this time.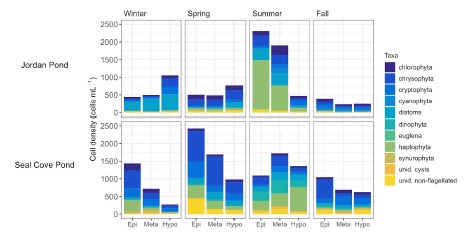
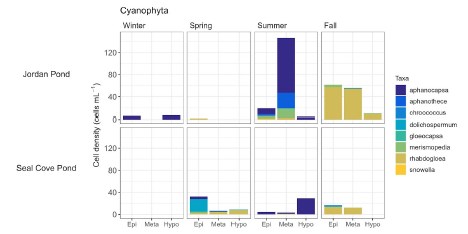
Historical Perspectives
The Air & Water Quality program at ANP has provided a rich set of lake data from 1995 to 2020, i ncluding Secchi depth, chlorophyll a concentration, DOC concentration, TP, TN, and TN:TP (Figure 16). The 25%, 50%, and 75% quantile regression values listed in Table 2 can be used as a historical baseline against which to compare contemporary values of Secchi depth, chlorophyll a , DOC, and nutrients. Sustained values surpassing 75% quantiles of a given metric or combination of metrics might provide early warning signals that a lake is approaching a threshold that could support HAB formation and development. We will refer to the historical data from Table 2 throughout the Discussion as we contextualize the 2020 sampling results of this project. Summer DOC sampling for the Northeast Temperate Network did not begin until 2012, creating a gap in DOC concentration data from 2006-2012.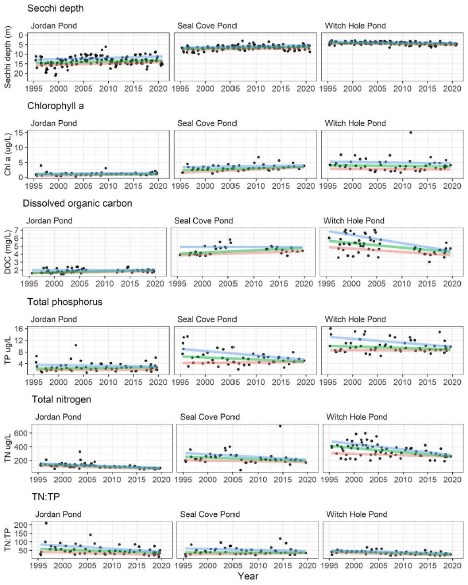
25% quantiles
Lake | Secchi (m) | Chl a (µg L -1 ) | DOC (mg L -1 ) | TP (µg L -1 ) | TN (µg L -1 ) | TN:TP |
---|---|---|---|---|---|---|
Jordan Pond | 17.6 | 0.8 | 1.7 | 1.9 | 90 | 31.4 |
Seal Cove Pond | 8.5 | 2.5 | 4.0 | 4.2 | 200 | 32.4 |
Witch Hole Pond | 3.7 | 2.7 | 4.3 | 8.6 | 273 | 28.1 |
50% quantiles
Lake | Secchi (m) | Chl a (µg L -1 ) | DOC (mg L -1 ) | TP (µg L -1 ) | TN (µg L -1 ) | TN:TP |
---|---|---|---|---|---|---|
Jordan Pond | 15.8 | 1.0 | 1.7 | 1.9 | 120 | 43.2 |
Seal Cove Pond | 8.0 | 3.0 | 4.0 | 4.5 | 220 | 38.4 |
Witch Hole Pond | 3.7 | 3.7 | 4.3 | 4.8 | 340 | 35.0 |
75% quantiles
Lake | Secchi (m) | Chl a (µg L -1 ) | DOC (mg L -1 ) | TP (µg L -1 ) | TN (µg L -1 ) | TN:TP |
---|---|---|---|---|---|---|
Jordan Pond | 14.5 | 1.2 | 2.0 | 3.2 | 133 | 56.5 |
Seal Cove Pond | 7.6 | 3.7 | 4.9 | 7.0 | 264 | 54.0 |
Witch Hole Pond | 2.8 | 4.7 | 5.7 | 11.0 | 403 | 42.3 |
Meteorology
Average air temperature was highest in July, peaking at 26.8°C on 7/21/20 (Figure 17). Air temperature declined through late summer into the fall, and average air temperature was 15.2°C i n September. In Maine, 2020 was a drought year, and precipitation frequency and magnitude were low in the weather station data. Notable precipitation events occurred on 6/26/20 (13 mm), 6/29/20 (11 mm), 8/29/20 (25 mm), and 10/14/20 (27 mm). Mean and maximum wind speed were high at the beginning of June, then decreased through July and the beginning of August before increasing throughout the fall. Mean wind speed in June and September were 1.6 m s - 1 and 1.8 m s - 1 , respectively, and maximum wind speed reached 5.9 m s - 1 at the beginning of June and 5.7 m s - 1 on 8/23/20, followed by more days in the fall with similar maximum wind speed values, following the mean wind speed trend.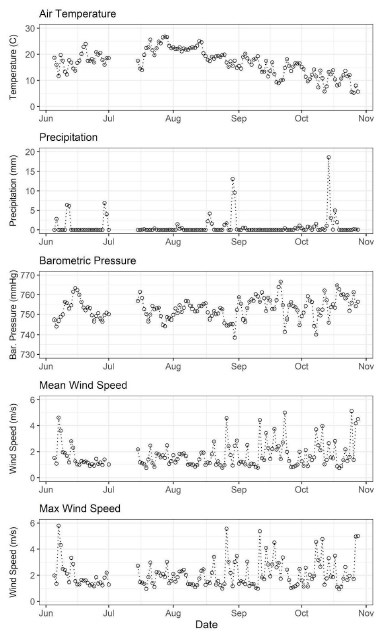
Lake Bathymetry
Jordan Pond (Figure 18) and Seal Cove Pond (Figure 19) are aligned on a north-south axis, and Witch Hole Pond (Figure 20) is tilted on a southwest-northeast axis. Mean depth of Jordan Pond i s 21.1 m, maximum lake depth is 49.3 m, and surface area is 75.9 ha. For Seal Cove Pond, mean lake depth is 4.0 m, maximum depth is 13.7 m, and surface area is 104.7 ha. Witch Hole Pond mean depth is 2.1 m, maximum depth is 10 m, and surface area is 9.7 ha.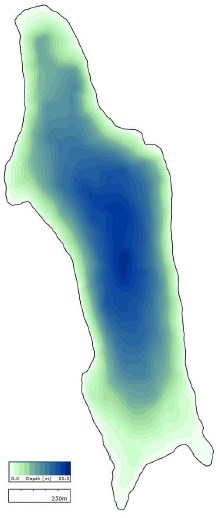
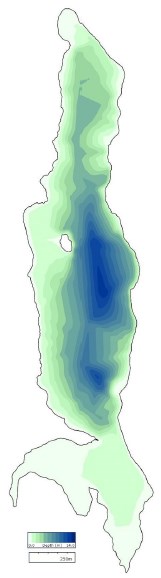
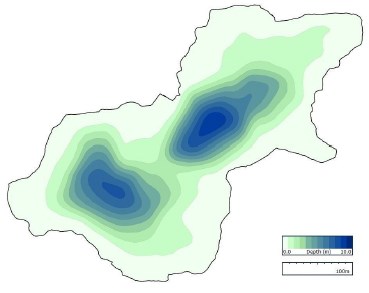
Risk Assessment Scorecard
Jordan Pond, Bubble Pond, Eagle Lake, Upper Breakneck Pond, and Long Pond (MDI) were categorized as having low risk for development of potential future HABs (Figure 21). Seal Cove Pond, Aunt Betty Pond, Lake Wood, Upper and Lower Hadlock Ponds, Echo Lake, Bear Brook Pond, and Lower Breakneck Pond were classified as moderate risk. Witch Hole Pond, Round Pond, Hodgdon Pond, and Seawall Pond were categorized as having considerable risk for future development of HABs. Of the six drinking water lakes in ANP, four were included in the “low risk” category (Bubble Pond, Eagle Lake, Jordan Pond, and Long Pond) and two were in the “moderate risk” category (Upper Hadlock Pond and Lower Hadlock Pond).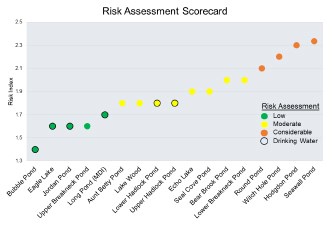
Discussion
Key Findings among Lakes: Temperature, Dissolved Oxygen, & Phycocyanin
Temperature and dissolved oxygen trends in Jordan Pond during the open water season of 2020 deviated from those of Seal Cove Pond and Witch Hole Pond. The epilimnetic temperature i n Jordan Pond remained lower than that of Seal Cove and Witch Hole Ponds, while the temperature of the bottom sensor (30 m depth) remained at approximately 6.6°C after stratification. The bottom sensor temperature measurements of Seal Cove Pond (10 m depth) and Witch Hole Pond (7 m depth) increased throughout the season to maxima of 20°C and 16°C, respectively.Dissolved oxygen in the epilimnion of Jordan Pond declined from 13 mg L - 1 at the beginning of the open water season to a minimum of 7 mg L - 1 at the end of August. Dissolved oxygen measured by the bottom sensor declined more gradually over the season, and was often greater than DO measured by the top sensor. In Seal Cove and Witch Hole Ponds, epilimnetic DO was similar to that of Jordan Pond, but DO measured by the bottom sensors declined rapidly throughout the season. Anoxia in the bottom water occurred from the last week in July to early September in SCP and from early July to late September in WHP.
Phycocyanin was generally low throughout the water column of Jordan Pond and Seal Cove Pond during the ice-free season, except for some isolated instances during the summer. Near the surface, however, phycocyanin peaked after ice-out and occurred again in July in Jordan Pond and at the end of September in Seal Cove Pond. In Witch Hole Pond, water column phycocyanin persisted at most depths throughout the ice-free season, particularly in the summer months. Water column measurements of phycocyanin were lower in WHP than surface phycocyanin peaks in JP and SCP.
Context for Findings: Roles in HAB Formation
Temperature and Thermal Stratification
Air temperature has increased substantially in the northeast United States over the last century (Vose et al. 2017), and climate models forecast sustained, rapid temperature increase in Maine (Fernandez et al. 2020). Therefore, it is likely that surface water temperature will continue to i ncrease in Maine lakes, as surface water temperature has been linked to air temperature (Paerl et al. 2008; Taranu et al., 2012; O’Reilly et al. 2015). Surface water temperature is a strong predictor of cyanobacterial blooms (Kosten et al., 2012; Persaud et al. 2015). In Canadian temperate lakes (Persaud et al. 2015), and a large study of lakes across a latitudinal gradient from Europe to South America (Kosten et al. 2012), cyanobacterial biovolume increased with water temperature. In a study of 200+ lakes in northeastern North America, Richardson et al. (2017) found that from 1975-2012, lakes had increasing surface temperatures and stronger thermal stratification. Lakes nearer to the Atlantic coast (<60 km) had cooling deepwater temperatures, causing greater density differences throughout the water columns. In a study of ~400 lakes from 1941-2017, Jane et al. (2021) found that strength of thermal stratification has increased in 84% of lakes that stratify.
As in Pilla et al. (2018), Richardson et al. (2017) described trends of near-surface water temperatures of northeastern North American lakes rising faster than air temperature, suggesting that our region may be a warming hotspot for lakes, similar to areas around the Laurentian Great Lakes and northern Europe (O’Reilly et al. 2015). These lakes may be changing more quickly than lakes in other mid-latitude regions due to amplified climate effects (i.e. warmer temperature with more precipitation; Richardson et al. 2017). The clearest lakes in the study (Secchi >5m) warmed the most rapidly and experienced the greatest increases in thermal stratification strength (Richardson et al. 2017).
Cyanobacteria generally dominate at high temperatures as a result of physiological and physical factors (O’Neil et al. 2012). Warmer water reduces the density of the epilimnion, thus strengthening vertical stratification (Paerl & Huisman 2009), and cyanobacteria typically experience optimal growth rates in warmer water, >25°C (Robarts & Zohary 1987; Coles & Jones 2000; Paerl & Huisman 2009). With increasing water temperature and limited wind mixing, the water column becomes stagnant and buoyant cyanobacteria move upward through the water column, sometimes leading to surface blooms and concentrations of toxins (Paerl & Huisman 2009). The viscosity of water is reduced as temperature increases (Hutchinson 1957), and according to Stokes Law, the vertical velocity of small organisms (like cyanobacteria) are i nversely proportional to viscosity (Reynolds 2006). Therefore, increasing water temperature reduces resistance to upward vertical migration by buoyant cyanobacteria. This process allows competitive advantage of buoyant cyanobacteria over diatoms in stratified water (Jöhnk et al. 2008; Paerl & Huisman 2009).
In lakes, warm winters can influence subsequent seasonal succession, through the lakes’ “ecological memory” (Anneville et al. 2015). Padisak (1992) described ecological memory as the capacity of an ecosystem or its past states to influence current or future responses of the community. For example, Anneville et al. (2015) found an autumn exceptional hot weather period (EHWP) promoted cyanobacteria growth in mesotrophic lakes and increased cyanobacterial biomass, but did not change the composition of the phytoplankton community, indicating that warmer autumns benefit the entire phytoplankton community. The EHWP did not affect an oligotrophic lake, perhaps because P concentration was too low to support high phytoplankton biomass (Anneville et al. 2015). At high temperatures in eutrophic lakes, stable water columns can promote cyanobacterial blooms (Paerl 1988; Zhang & Prepas 1996). The i nteraction of water temperature and lake trophic state is a key factor in explaining phytoplankton biomass (Rigosi et al. 2014, Kraemer et al. 2017). Reinl et al. (2023) described how cyanobacterial blooms can also occur during cold water conditions, even when lakes are ice-covered. According to Wetzel (2001), most lakes are located in regions where water temperatures are <15°C for at least half the year. Many cyanobacterial taxa are adapted to cold-water conditions, and while bloom-forming cyanobacteria are often viewed as r -selected opportunists that can outcompete diatoms, chlorophytes, and other eukaryotes only during high temperatures during the stratified period, cyanobacteria actually fill a wide range of niches and individual taxa can develop blooms during cold periods as winners of K -selection (Reinl et al. 2023). For example, in November 2020, Aphanizomenon sp. formed a surface scum in Salmon Lake, ME, and late-fall blooms of Microcystis have also occurred in New York and Vermont after fall turnover (Reinl et al. 2023).
The maximum temperature recorded by the top miniDOT sensor in Jordan Pond was 24.9°C on 8/14/20. Surface temperatures in Seal Cove Pond and Witch Hole Pond reached >28°C at the end of July and beginning of August, respectively, and both lakes experienced temperatures 25°C for 4-5 weeks spanning the second half of July into the third week of August. Data from the Jordan Pond Buoy Project and ANP Air & Water Quality program show that average August surface water has increased almost 2°C since 2013, while the annual average temperature of the hypolimnion has increased approximately 2°C (Gawley 2023). Similar increases have occurred in Seal Cove Pond, with average epilimnion temperature rising from 18°C in 2006 to 20°C in 2018. In Witch Hole Pond, average epilimnion temperature (May-September) increased from 18.5°C in 2006 to 21°C in 2022. If cyanobacteria typically experience optimal growth in water temperature >25°C (Robarts & Zohary 1987; Coles & Jones 2000; Paerl & Huisman 2009), Seal Cove Pond and Witch Hole Pond may be most vulnerable to cyanobacterial blooms, particularly from the third week in July to the third week in August. Long-term water temperature trends suggest that average surface water temperatures in all three lakes are increasing steadily, with the greatest long-term increase in epilimnion temperature occurring in Witch Hole Pond. If present in the lakes, buoyant cyanobacterial taxa like Microcystis and Dolichospermum may have a competitive advantage over other phytoplankton groups during periods of high epilimnetic temperature.
Dissolved Oxygen (DO)
“Oxygen is the most fundamental parameter of lakes and streams, aside from water itself”(Wetzel 2001). In eutrophic waters, DO is a precursor for cyanobacterial blooms (Trimbee & Prepas 1988), especially when water temperature is high and the water column is stable, during the period of summer stratification. With oxygen depletion in bottom water, lake sediments can release P, which benefits buoyant cyanobacteria taxa. Increases in water temperature stimulate decomposition of organic matter and microbial respiration and decrease oxygen solubility (Hutchinson 1957; Blumberg & Di Toro 1990; Jenny et al. 2016). Generally, the prevalence and duration of hypolimnetic anoxia are increasing with climate change effects and land use change (Jenny et al. 2016; Jane et al. 2021; Carey et al. 2022). However, increasing severity and frequency of storms can increase lake water mixing and oxygen availability throughout the water column (Prein et al. 2017), so there may be large daily fluctuations in DO concentration (Perello et al. 2017; Carey et al. 2022).
Hypolimnetic dissolved oxygen concentration influences internal nutrient loading (Pettersson et al. 2003; Adrian et al. 2009)—DO depletion in the hypolimnion can prompt the release of P (Nürnberg 1984; Prepas & Vickery 1984) and ammonium (Wetzel 2001) into the water column (Foley et al. 2012). Furthermore, Fe may undergo reduction during anoxic conditions, leading to release from sediments as Fe 2+ , which is more biologically available. Algal blooms can lead to anoxic bottom-water conditions, creating a positive feedback cycle that stimulates nuisance blooms, especially those created by buoyant taxa that can move from nutrient-rich bottom waters to oxygen-rich surface waters (Paerl et al. 2001; Huber et al. 2012; Rigosi et al. 2014; Wagner & Adrian 2009; Paerl et al. 2018). Additionally, increases in the duration of the stratification period isolate bottom water from access to atmospheric oxygen for longer periods (Foley et al. 2012). Therefore, poorly flushed lakes that experience strong thermal stratification promote hypoxic or anoxic conditions and may be more likely to experience harmful algal blooms (Paerl et al. 2001). When lake mixing occurs, the accumulated P is transported to the epilimnion (Riley & Prepas 1984), potentially promoting algal growth (Trimbee & Prepas 1988).
Trimbee & Prepas (1988) found that even without sediment P release, the onset of anoxic conditions (<2 mg L - 1 ) over lake sediments could boost the proportion of cyanobacteria biomass i n the phytoplankton community. Anoxic conditions over lake sediments favor recruitment of benthic overwintering colonies or filaments of cyanobacteria (Reynolds et al. 1981; Trimbee & Harris 1984). Basin morphometry also plays an important role in anoxia (Molot et al. 2014). Recruitment of cyanobacteria decreases with lake depth, and also with the proportion of lake sediment overlain by oxic water (Trimbee & Prepas 1988). These findings suggest that the timing and extent of oxygen depletion of bottom waters can be used as predictors of timing and magnitude of harmful algal blooms in north temperate lakes (Trimbee & Prepas 1988; Jane et al. 2021).
While anoxia did not occur in deep Jordan Pond, bottom-water anoxia was present from late July to early September in Seal Cove Pond, and from early July to late September in Witch Hole Pond. These anoxic conditions, of longest duration in Witch Hole Pond, may favor recruitment of benthic overwintering cyanobacteria. As recruitment decreases with lake depth (Trimbee & Prepas 1988), Seal Cove Pond and Witch Hole Pond may be more likely to have sedimentary cyanobacterial recruitment. Bottom-water anoxia in Seal Cove Pond and Witch Hole Pond could act as a precursor to peaks in cyanobacterial biomass, as observed in a north temperate stratified lake in mid-late August (Trimbee & Prepas 1988).
Zhao et al. (2019) found that cyanobacterial blooms correlated to DO levels of 5.0 to 8.3 mg L −1 i n temperate lakes and reservoirs in China. These levels are higher than DO measurements from bottom sensors in Seal Cove and Witch Hole Ponds from most of the stratified season, as well as top waters from late-June onward in Witch Hole Pond, July onward in Seal Cove Pond, and in Jordan Pond during parts of August and September.
Dissolved oxygen had complicated seasonal patterns in other north temperate lakes (Hanson et al. 2006). Peaks in DO occurred shortly after ice-off and before ice-on, and minima occurred in l ate-winter and during the summer. Such annual patterns show that DO is mediated on a seasonal scale by biological processes in addition to fluctuations in temperature (Hanson et al. 2006, Jane et al. 2021). For example, Hanson et al. (2006) showed that DO minima have occurred 10 days after peak water temperature. Wong & Hobbs (2020) described seasonal trends in water temperature and DO increasing in early summer and peaking in August to early September, indicating increased photosynthetic activity during warmer periods that are characteristic of an algal bloom. In mid-September, DO declined as the algal bloom dissipated.
The DO patterns reported by Wong & Hobbs (2020) in a lake that experiences algal blooms are not evident in Jordan Pond, Seal Cove Pond, or Witch Hole Pond. Instead of increasing with temperature, peaking in August to early September, and declining in mid-September, epilimnetic DO in all three lakes had an inverse relationship with temperature, reaching minima during the summer and gradually increasing after mid-September. As in Hanson et al. (2006), epilimnetic DO minima in ANP lakes occurred after peak water temperature—14 days for Jordan Pond, 19 days for Seal Cove Pond, and 15 days for Witch Hole Pond. These results suggest that DO in Jordan Pond, Seal Cove Pond, and Witch Hole Pond is regulated by biological processes as well as physical processes (such as temperature), but that the role of biological processes (e.g. algal primary productivity) is weaker than in the lake described by Wong & Hobbs (2020) that regularly experiences cyanobacterial blooms.
Anoxic conditions are common in Maine lakes during peak stratification (8/1 to 9/7; Deeds et al. 2021). Anoxia was observed in 647 out of 951 Maine lakes surveyed (ME DEP 2015). However, many lakes in Maine have low epilimnion TP concentrations during peak stratification (median 9.0 µg L - 1 ), and may be predisposed to anoxia resulting from natural conditions, as opposed to anthropogenic nutrient enrichment (Deeds et al. 2021). Water clarity also contributes to trends in DO. In lakes with low clarity (Secchi <2 m), DO increased when the average mean summer surface water temperature exceeded 24°C (Jane et al. 2021). After blooms occur in eutrophic l akes, deep-water DO is generally very low (median 0.64 mg L - 1 , relative to 3.42 mg L - 1 i n other l akes) due to sinking and decomposition of algal biomass in bottom-water habitats (Jane et al. 2021). Like other Maine lakes described by Deeds et al. (2021), the bottom waters of Seal Cove Pond and Witch Hole Pond became anoxic during peak stratification. However, Secchi depth is greater than 2 m in all three lakes, so clarity may not be a strong driver of DO in these lakes.
Nutrients & Dissolved Organic Carbon
The role of nutrients in promoting harmful algal blooms is well documented, with evidence that i n freshwater North American and European lakes, P is the main driver of algal blooms (Schindler 2012; Schindler et al. 2016), or that both N and P are instrumental in HAB development (Paerl et al. 2001; Watson et al. 2015). For example, lakes with TP <5 µg L - 1 generally don’t support HABs (Downing et al. 2001; Schindler et al. 2012). Within-lake nitrogen-fixation, even in eutrophic lakes, however, may be too low to sustain ecosystem N requirements, so N inputs to lakes may play a key roles in HAB development of some systems (Paerl et al. 2018). The U.S. EPA Nutrient Ecoregion 8 (including the entire states of Maine, New Hampshire, and Vermont) nutrient criteria for lakes are TP ≤8 µg L - 1 and TN ≤240 µg L - 1 (U.S. EPA 2000a). These values represent levels that protect lakes from over-enrichment of nutrients from cultural eutrophication and maintain quality of water resources (Gawley & Wiggin 2016).In lakes, delivery of P via short-term and seasonal loading events is important for primary productivity and formation of HABs (Pearl et al. 2001). Discharge of P to lakes can occur via surface runoff during spring melt or in acute pulses from precipitation events (Paerl et al. 2001), and can be introduced by anthropogenic activities, especially in lakes that are not protected from anthropogenic inputs. In lakes, TP is regulated by geology and hydrology of the watershed. Basin morphometry can also induce naturally-occurring anoxia (Deeds et al. 2021). While N exists in several dissolved and particulate forms in lakes, and can be transformed to aqueous and gaseous forms that are biologically available and can be exchanged between the atmosphere, water column, and sediment, P exists in fewer biologically available aqueous forms (Paerl et al. 2001).
In 2020, average epilimnetic TP and TN were similar to or lower than the long-term averages (1995-2020) in all three study lakes. TN in Seal Cove Pond and Witch Hole Pond were lower than or equal to 25% quantiles of long-term averages (ANP Water Quality data). Average epilimnetic TP was <5 µg L - 1 i n both Jordan Pond (2.7 µg L - 1 ) and Seal Cove Pond (4.7 µg L - 1 ), and was 9.2 µg L - 1 i n Witch Hole Pond during the stratified period. Average epilimnetic TN was <240 µg L - 1 i n Jordan Pond (96 µg L - 1 ) and Seal Cove Pond (200 µg L - 1 ), and was 259 µg L - 1 i n Witch Hole Pond. Even though TP and TN concentrations in Witch Hole Pond were high enough to support HABs and surpass the EPA nutrient criteria for lakes in 2020, average epilimnetic TP and TN in Witch Hole Pond were similar to the 1995-2020 long-term averages.
During anoxic conditions, P is predicted to be released from the sediment if the sediment ratio of aluminum (Al) to iron (Fe) is <3 and if Al:P is <25 (Kopacek et al. 2005). While Jordan Pond meets these criteria (Kristin Strock, unpublished data), we do not have evidence that Jordan Pond bottom waters become anoxic, even during peak stratification. Seal Cove Pond does not meet these criteria, so anoxic bottom waters likely do not prompt internal P loading from sediment release. The more likely source of P in Seal Cove Pond is influx from the watershed during spring and early summer. We do not have sediment chemistry data on the Al:Fe and Al:P ratios for Witch Hole Pond, but our nutrient data suggest that anoxic conditions are triggering the release of sediment P to the water column, in addition to watershed inputs.
Eutrophic, P-enriched lakes with N:P<15 are expected to be more susceptible to diazotrophic (nitrogen-fixing) cyanobacterial taxa like Aphanizomenon, Dolichospermum, and Cylindrospermopsis , while lakes with N:P>20 are more susceptible to non-nitrogen fixing taxa like Microcystis and Planktothrix (Schindler 1977; Smith et al. 1999; Havens et al. 2003; Watson et al. 2015). Watson et al. (2015) describe how seasonal succession of bloom-formers with different environmental optima can occur in lakes with shifting N dynamics. After biologically available N is drawn down by spring blooms of diatoms and green algae, N-fixing cyanobacterial taxa increase, and may then be succeeded by non-N-fixing cyanobacterial taxa (Paerl et al. 2018). Microcystis, Dolichospermum , and Cylindrospermopsis are highly competitive for dissolved inorganic P and also have the ability to acquire P from organic compounds (O’Neil et al. 2012). With wide availability of N sources, these taxa can dominate during eutrophic conditions, but also when concentrations of N and P are low, due to their competitive advantages (O’Neil et al. 2012). In Jordan Pond, Seal Cove Pond, and Witch Hole Pond, long-term annual epilimnetic TN:TP>20, suggesting that if cyanobacterial blooms occurred in these lakes, they might be composed of non-N-fixing taxa like Microcystis.
Because N and P are both generally low in northern lakes, researchers have highlighted the i mportance of dissolved organic matter (DOM) as a driver of HAB formation (Sorichetti et al. 2014; Creed et al. 2018). Many cyanobacterial taxa can use DOM as a nutrient source (Paerl et al. 2001). Additionally, increases in DOM can alter light dynamics in lakes by absorbing UV radiation and decreasing the depth of photosynthetically active radiation (PAR; Finstad et al. 2014). These conditions may select for positively buoyant cells, like cyanobacteria (Creed et al. 2018).
Increasing DOC concentration in brown-water lakes is also associated with warmer epilimnia and cooler hypolimnia (Pilla et al. 2018), which may increase oxygen concentration in the hypolimnia (Nelligan et al. 2019). However, lakes with higher DOC concentrations (e.g. >10 mg L - 1 ; Couture et al. 2015) also experience greater microbial oxygen demand (Clilverd et al. 2009) and thermal stability (Fee et al. 1996; Pilla et al. 2018), so brown-water lakes may experience more frequent and longer low-oxygen conditions (Brothers et al. 2014; Couture et al. 2015).
In 2020, average epilimnetic DOC concentrations in Jordan Pond, Seal Cove Pond, and Witch Hole Pond were relatively low: ≤4 mg L - 1 . These concentrations are similar to or lower than the l ong-term 1995-2020 averages. DOC concentrations are consistently greater and Secchi depths are shallower in browner Seal Cove Pond and Witch Hole Pond compared to clear Jordan Pond. In a comparison of algal dynamics over the last two centuries in browner Seal Cove Pond versus clearer Jordan Pond, Fowler et al. (2022) found evidence of more and different types of cyanobacterial pigments in Seal Cove Pond than in Jordan Pond. Terrestrial subsidies of DOC to Seal Cove Pond may have contributed to these algal dynamics.
Chlorophyll a
While measures of the pigment chlorophyll, an indicator of algal biomass, are sometimes correlated with phycocyanin fluorescence (Kasinak et al. 2015; Wong & Hobbs 2020), chlorophyll may not accurately represent the cyanobacterial population (Rigosi et al. 2014). Total chlorophyll a and cyanobacterial biomass can differ in response to environmental variables like temperature and nutrients (Elliott et al. 2006).In a whole-lake experiment, Buelo et al. (2022) used a lake-specific threshold of 14 µg L - 1 chl a to mark the date of algal bloom onset in a small oligotrophic lake in Michigan (46°N), based on region-specific historical data from Wilkinson et al. (2018). In this lake, the timing of the algal bloom, indicated by chl a measurements, coincided with a peak in phycocyanin fluorescence, as well as a peak in dissolved oxygen (Buelo et al. 2022). In the same lake, Pace et al. (2017) documented simultaneous increases in chlorophyll concentration and phycocyanin fluorescence, with a chlorophyll max near 40 µg L - 1 occurring at the end of June. While there is not a specific threshold that defines algal bloom conditions across all lakes, chlorophyll a concentration of ~20 µg L - 1 corresponds to trophic state index (TSI) of 60, indicative of highly enriched lake conditions (Carlson & Simpson 1996; Pace et al. 2017).
During peak stratification in 2020, average epilimnetic chlorophyll a concentrations were ≤4 µg L - 1 , below the values given in the studies above as l ake-specific thresholds for algal blooms. Compared to long-term trends (1995-2020), chlorophyll a concentration was similar to the average in Jordan Pond, below the 25% quantile (below average) in Seal Cove Pond, and between the 50% and 75% quantiles (above average) in Witch Hole Pond. Chlorophyll fluorescence was generally low on the surface and in the water column of Jordan Pond, and higher in the water column of Seal Cove Pond in the spring and early summer. Witch Hole Pond had the highest chlorophyll fluorescence in the water column, particularly from mid-summer to early fall, during the anoxic period. Increases in algal biomass during this time may have been fueled by internal P loading. DIN:TP data from 2020 suggest that Witch Hole Pond was more l ikely to have been N-limited in the summer, but that the hypolimnion was P-limited during the fall.
Basin Morphometry
In lakes with TP <15 µg L - 1 , basin morphometry may be a primary driver of areal anoxia and dissolved oxygen depletion rates (Molot et al. 1992; Molot et al. 2014). The morphometric ratio of a lake (mean depth:(surface area) 0.5 ; Osgood 1988) i s positively correlated to anoxia in stratified lakes (Nürnberg 1995). Lakes with a high morphometric ratio are generally more strongly stratified (Foley et al. 2012). The morphometric ratio of Jordan Pond is 2.4, while Seal Cove Pond and Witch Hole Pond have much smaller morphometric ratios, of 0.4 and 0.7, respectively. Consistent with findings of Molot et al. (2014) and Nürnberg (1995), seasonal anoxia occurred in Seal Cove Pond and Witch Hole Pond, and not Jordan Pond.With a model using data from >400 Maine lakes, Deeds et al. (2021) found that basin morphometry and thermal stratification are the most important drivers of anoxia in low-nutrient temperate lakes in Maine. Specifically, maximum lake depth and proportion of total lake area under the epilimnetic mixed layer during peak stratification were the greatest predictors of hypolimnetic anoxia. The likelihood of hypolimnetic anoxia increases with decreasing maximum l ake depth and increasing lake area beneath the mixed layer (Deeds et al. 2021).
Maximum depth is correlated to lake volume—deeper and more voluminous dimictic lakes hold more dissolved oxygen and lose relatively less hypolimnetic oxygen before fall turnover restores DO throughout the water column due to larger DO reserves and greater thermal stability (Deeds et al. 2021). In lakes with large shallow areas with a high proportion of sediment in contact with epilimnetic waters (small area under mixed layer), dissolved oxygen may be regularly replenished from surface mixing with the epilimnion. In contrast, lakes with smaller shallow areas in contact with the epilimnion (large area under mixed layer), are more likely to experience depletion of DO without replenishment from the epilimnion (Deeds et al. 2021). Lakes that are shallower, with large catchment area to lake area ratios, tend to be darker in color with higher TP concentrations (Webster et al. 2008).
Like other large lakes described by Deeds et al. (2021), Jordan Pond is deep and voluminous, and has high hypolimnetic DO throughout the stratified period, likely due to strong thermal stratification and large reserves of DO. Seal Cove Pond and Witch Hole Pond are shallower, with larger catchment area to lake area ratios than Jordan Pond. Similar to the lakes in Webster et al. (2008), they are also darker in color with higher TP concentrations than Jordan Pond. The morphometric characteristics of Jordan Pond make it less susceptible to anoxic conditions than those of Seal Cove Pond or Witch Hole Pond, which could lead to reduced risk of HAB development.
Meteorological Variables
In addition to lake and watershed variables, harmful algal blooms are also driven by meteorological conditions like air temperature (Paerl & Huisman 2008; Kosten et al. 2012; Taranu et al. 2012), precipitation (Reichwaldt & Ghadouani 2012; Romo et al. 2013), and wind speed and direction (Kanoshina et al. 2003; Cao et al. 2006). These variables affect thermal stratification, primary production, dilution, PAR, and sediment and nutrient dynamics (Zhang et al. 2021). Persaud et al. (2015), in a forecasting study of cyanobacterial dominance in Canadian temperate lakes, summarize how effects of climate change on meteorological variables may i ncrease the geographical extent, seasonal duration, and frequency of cyanobacterial blooms (Jöhnk et al. 2008; Paerl & Huisman 2009; Persaud et al. 2014).These meteorological variables may be especially insightful for evaluating inter-annual trends in cyanobacterial bloom intensity, duration, and timing (Persaud et al. 2014).Air Temperature
While air temperature is broadly linked to surface water temperature globally, air and lake temperature trends diverged for many individual lakes from 1985-2009, as surface water warming rates depend not only on regional climate, but local characteristics (O’Reilly et al. 2015). On average, surface water temperatures of ice-covered lakes are warming more quickly than lakes with no ice cover, at a rate of 0.48°C decade - 1 (O’Reilly et al. 2015). In Scandinavian l akes, cyanobacterial biomass increased in spring and early summer due to warmer winter temperatures (Weyhenmeyer 2001). Anneville et al. (2015) also found that warm winters were associated with increased cyanobacterial abundance. The European heat wave of 2003 triggered a four-fold increase in cyanobacterial abundance in a northern European lake (Jöhnk et al. 2008). The increased stability of the water column resulting from high temperatures reduces vertical mixing, favoring buoyant cyanobacteria (Khan et al. 2015), including toxic strains like Microcystis aeruginosa (Watson et al. 2015). Ho & Michalak (2020), using 1200 observations, found that summer temperatures drove total phytoplankton abundance and were associated with increased TP concentration, and also that high temperatures may reduce the toxicity of cyanobacterial blooms. For example, warmer spring air temperature was associated with lower microcystin concentrations, even while cyanobacterial abundance increased. Other researchers have also found that longer summers may result in shifts toward more nontoxic cyanobacterial taxa (Anneville et al. 2015; Peng et al. 2018).From 2013 to 2022, average summer air temperature at the Jordan Pond House weather station was 17.5 ± 0.7°C. Summers of 2020 and 2021 were particularly warm, with average air temperatures of 18.4°C and 18.5°C, respectively. Average summer air temperature did not directly mediate surface water temperature at Jordan Pond, but August air temperature had a clearer effect on surface water temperature during a period from 2017 to 2022 (R 2 =0.86, n=5). In August 2018, for example, average air temperature was 21.3°C and average surface water temperature was 24°C, while in August 2020, average air temperature was 20.1°C and average surface water temperature was 22.8°C. These results suggest that August air temperature closely controls surface water temperature in Jordan Pond, and likely other lakes in ANP. It is unlikely that air temperature effects on surface water temperature were responsible for higher phycocyanin fluorescence measurements in Jordan Pond surface waters during 2020, as these measurements occurred prior to maximum summer temperatures.
Precipitation
Increases in precipitation can reduce water clarity by transporting DOM from the landscape to lakes (Hongve et al. 2004; Couture et al. 2012; Williamson et al. 2014; Williamson et al. 2015; Rose et al. 2016). In the northeastern United States, large increases in precipitation intensity and severity have occurred since 1901 (Easterling et al. 2017), and heavy precipitation events are expected to increase in Maine (Fernandez et al. 2020), particularly in summer months (Prein et al. 2017). Severe precipitation events can change water pathways through watersheds and i ncrease leaching of DOM from the upper soil layer (Hongve et al. 2004), while also changing the character of DOM in inland waters (Murshed et al. 2014). Effects of seasonal precipitation on DOM character have been described for lakes in ANP—increases in UV-absorbing DOM were most apparent for lakes with a large watershed area to lake area ratio after an autumn precipitation event (Warner et al. 2020). Intense precipitation events can also increase vertical mixing in lakes and reduce stability (Kimura et al. 2014).Increases in storms may or may not promote harmful algal blooms in lakes, as allochthonous nutrient delivery increases, but water residence time and stratification strength decrease (Paerl & Huisman 2009; O’Neil et al. 2012; Ho & Michalak 2020; Reinl et al. 2021). Warner & Saros (2019) found evidence of increased nutrients and chlorophyll a concentration after precipitation events in Maine drinking water lakes. In a large study of oligotrophic to eutrophic lakes across the Northeast and Midwest United States, Wilkinson et al. (2021) described a marginal positive trend in algal bloom magnitude in lakes experiencing intensified precipitation. If increases in late winter/early spring precipitation are followed by drought, as was observed between the mid-1990s to mid-2000s, blooms are more likely to occur (Paerl & Huisman 2009), as many toxin-producing cyanobacterial taxa are able to exploit and store nutrients (Jöhnk et al. 2008). Factors like light and temperature will be important determinants of bloom development. Greater nutrient limitation in oligotrophic lakes may favor cyanobacterial dominance (Reinl et al. 2021), particularly if nutrient pulses are accompanied by increased temperature (Lürling et al. 2018). Interactions between temperature and hydrologic conditions alter the likelihood of cyanobacterial bloom development (Jöhnk et al. 2008; Paerl & Paul 2012), so even if individual precipitation or temperature events are not considered extreme, simultaneous changes in both variables could create an “extreme” condition with implications for cyanobacterial bloom development (Khan et al. 2015).
At the Jordan Pond House weather station in 2020, average daily summer precipitation was 0.6 mm, compared to 1.2 ± 0.6 mm, the average daily summer rainfall from 2013-2022. In 2020, DOC concentration, nutrient levels, and chlorophyll a in Jordan Pond, Seal Cove Pond, and Witch Hole Pond were generally below the 1995-2020 average (with the exception of Witch Hole Pond chlorophyll a, which was slightly above the long-term average). Reduced DOC, nutrient, and chlorophyll a concentrations may reflect l ower rainfall during the summer of 2020. These results agree with findings from Warner & Saros (2019), that precipitation can affect nutrients and chlorophyll a in Maine lakes. In rainier years, there may be increased export of DOM and nutrients to ANP lakes, potentially increasing the magnitude of algal blooms as described in Wilkinson et al. (2021), especially if paired with increased temperature (Lürling et al. 2018).
For ANP lakes, the ratio of catchment area to lake area (CA:LA), catchment slope, and catchment land cover type all contribute to terrestrial DOC transport to lakes after extreme precipitation events (Fowler, unpublished data). DOC transport to ANP lakes after extreme precipitation events is greater for lakes with shallower catchment slope, as low-relief watersheds tend to contain greater woody wetland area, which provides more DOC to lakes than any other l and cover type in ANP. Lakes with high CA:LA also experience larger pulses in DOC concentration after storm events, as proportionally greater inputs of DOC from the catchment affect relatively smaller lake basins. Watersheds with more deciduous, evergreen, and wetland l and cover provide more DOC to lakes than those with higher proportions of shrub-scrub, herbaceous plants, bare ground, or developed areas (Fowler, unpublished data). This means that Witch Hole Pond, with a CA:LA of 10 and a low-relief watershed, is likely to experience proportionately more terrestrial DOC transport after a storm than Seal Cove Pond (CA:LA = 7.4), and especially Jordan Pond, with its relatively steep catchment area and a lower CA:LA of 6.
Wind
The most recent IPCC report compiled several studies that suggest wind-speed decline, or stilling, is occurring globally at mid-latitudes (Seneviratne et al. 2021). While wind doesn’t determine algal biomass of lakes, it can contribute to formation and severity of blooms through i mpacts on vertical distribution of algae, particularly in eutrophic lakes (Zhang et al. 2021). Zhang et al. (2021) identified a threshold wind speed of 3 m s - 1 for the vertical distribution of chlorophyll a concentration in three lakes, with a negative correlation between wind speed and floating algal bloom area. Algal bloom area doubled when wind speed declined from 3 m s - 1 to 1 m s - 1 . In this study, a shallow lake was more sensitive to reductions in wind speed. This relationship suggests that with declining wind speeds, algal blooms may intensify in coming decades.Lake bathymetry and wind direction data may provide clues about lake areas most susceptible to blooms, with blooms expected to occur in downwind coves (Wynne et al. 2011; Zhang et al. 2021). In deep lakes, reductions in wind speed may lead to earlier onset and/or longer duration of stratification, with implications for nutrient exchange with surface waters and primary productivity. Reduced wind speed in shallow lakes may also promote earlier stratification, with effects on nutrient dynamics partly depending on the light environment (Zhang et al. 2021). Seasonality is also important—greater wind speeds during summer months may have less i mpact due to the strength of thermal stratification, but in the fall, windy days may speed up turnover, promoting bloom conditions as nutrients from bottom waters are mixed through the water column (Zhang et al. 2021).
The effects of wind on lake thermal dynamics are greater in large lakes than lakes with less surface area (<5 km 2 ), however (Fee et al. 1996). Jordan Pond, Seal Cove Pond, and Witch Hole Pond are far below this threshold. For reference, the entire watershed area for Jordan Pond is about 5 km 2 . In smaller lakes, water transparency i s a larger driver of thermal structure than wind (Read et al. 2012). Jordan Pond (and several other lakes in ANP) have relatively long fetches, however, due to their long and narrow shape, a result of glacial movement across the l andscape. In these lakes, strong winds might weaken stratification and/or deepen the thermocline, while reductions in wind could have the opposite effect, leading to a shallower thermocline with more stable stratification, favoring buoyant cyanobacteria (Jöhnk et al. 2008).
In the summer of 2020, mean (1.6 m s - 1 ) and maximum wind speed (1.6 m s - 1 ) at the Jordan Pond House weather station were similar to the 2013 to 2022 averages, 1.7 ± 0.1 m s - 1 and 2.1 ± 0.1 m s - 1 , respectively. Wind speed remained relatively unchanged during this period. These wind speeds are below the 3 m s - 1 threshold described by Zhang et al. (2021), indicating that wind speed could contribute to algal bloom area. However, the lakes in Zhang et al. (2021) were all eutrophic, in contrast to the lakes in our study, so it is reasonable to expect that relatively low wind speed in ANP would have much less impact on bloom area if an algal bloom were to develop in any of the lakes. However, low wind speed might be more likely to support earlier stratification in a shallow lake like Witch Hole Pond than in a deep lake like Jordan Pond.
During the period of stratification, wind in ANP often comes from the south and/or west. As Jordan Pond (Figure 18) and Seal Cove Pond (Figure 19) are aligned on a north-south axis, they may be more vulnerable to formation of cyanobacteria surface scums on shallow northern or eastern edges. The shallow east edges of the northern and southern basins in Seal Cove Pond might be particularly susceptible as they are bottle-necked from the deeper and wider central basin. Witch Hole Pond (Figure 20), smaller in surface area and shallower, is tilted on a southwest-northeast axis, and is likely more vulnerable to surface scums of cyanobacteria on its northern and eastern edges.
Ice-Out Timing & Seasonality
Lakes in Maine are sensitive to warm winter and spring temperature, and ice-out in Maine lakes is linked to winter degree day thresholds (Beyene & Jain 2015). Temporal synchrony in ice-out of Maine lakes is high, and ice-out has been occurring earlier in the year since the mid-20 t h century (Hodgkins et al. 2002; Boeff et al. 2016; Ellis & Greene 2019), and is related to the number of accumulated freezing and melting degree days in January and February (Beyenne & Jain 2015). Especially for lakes near the coast, such as those in ANP, the occurrence and magnitude of average monthly nonfreezing winter degree days has a strong effect on ice-out dates (Beyene & Jain 2015). Warner et al. (2018) described the effect of ice-out timing on thermal structure in Jordan Pond in ANP—with earlier ice-off, mixing depth in Jordan Pond was shallower. Ice-out trends in ANP lakes may affect HAB formation due to availability of nutrients, water temperature, and DO. For example, in 2012, early ice-out in Maine lakes led to a prolonged duration of thermal stratification, resulting in severe anoxia and P release from sediments. Lake-wide harmful algal blooms then developed in Lake Auburn and Georges Pond (Amirbahman et al. 2016).As described in Reinl et al. (2021), many oligotrophic lakes are located in higher latitudes with colder climates (Alin & Johnson 2007), where ice cover extent and duration is expected to decrease (Sharma et al. 2015). Temporal coherence in ice-off dates has been documented in Maine and New England (Hodgkins et al. 2002; Beyene & Jain 2015; Boeff et al. 2016) and the Laurentian Great Lakes region (Magnuson et al. 2005). O’Reilly et al. (2015) found that lake morphology is another important factor in the response of ice-covered lake warming to ambient air temperature. For example, the world’s deepest ice-covered lakes have warmed twice as fast as overlying air temperatures. In these lakes, shorter ice duration due to later freeze and earlier i ce break-up are affected by temperatures in preceding months (Magnuson 2000), and i ncreasing air temperature can lead to earlier onset of stratification and surface waters warming more rapidly than the air (Austin & Colman 2007). Surface water temperature more closely tracks air temperature in smaller, shallower lakes (Toffolon et al. 2014). Richardson et al. (2017) explained that for Northeastern North American (NENA) lakes and l akes in northern latitudes, warmer winters with less ice cover and less snow cover on ice will become more common (Magnuson et al. 2000; Hodgkins et al. 2002), lake albedo will decline, stratification onset will occur earlier, the duration of radiation absorption will lengthen, and summer warming will intensify (Austin & Colman 2007; O’Reilly et al. 2015; Zhong et al. 2016). Lakes at higher latitudes (i.e. >44°N in Richardson et al. 2017), experience the fastest increases i n the strength of thermal stratification and lake warming rates. Because higher latitudes are projected to have the greatest increases in air temperature warming on the coldest days (Seneviratne et al. 2021), lake surface temperatures in these areas may warm similarly, resulting in stronger density gradients between lake strata and changes in the phenology of ice dynamics, like earlier break-up (Richardson et al. 2017).
Changing winter weather will likely have important implications for oxygen dynamics. Warmer winter temperatures and earlier ice-off may have variable effects on hypolimnetic oxygen concentration (Nelligan et al. 2019). Earlier onset of summer stratification can stretch the period between seasonal mixing events and intensify oxygen depletion (Stainsby et al. 2011; Perello et al. 2017). However, earlier ice-off may reduce winter anoxia by shortening the duration of under-ice oxygen depletion (Agbeti & Smol 1994; Fang & Stefan 2009). Perello et al. (2017) monitored water temperature and DO to compare weather patterns with stratification and DO trends in Lake Erie, which has experienced declining lake ice since 1973 (Wang et al. 2012). In 2012, increased storm events corresponded to less ice coverage, warm water temperature, and l ow DO concentrations, with a deeper and thinner hypolimnion. Reduced ice cover allows for earlier wind mixing of the upper water layer, causing the hypolimnion to become deeper and thinner. In Maine lakes with lower DOC concentrations, it is likely that thermoclines will shallow as temperatures warm and ice-cover duration shortens. As the thermocline shallows, the area below the epilimnion will increase, and deep, low-DOC lakes will become more susceptible to anoxia (Deeds et al. 2021).
Risk Assessment
Early Warning Indicators
In multivariate, whole-ecosystem time series, like those of a lake over the course of one or several seasons, there are cases where temporal or spatial early warning indicators (EWIs) of cyanobacterial blooms, also called resilience indicators, have preceded breakpoints in ecosystem state variables (e.g. chlorophyll a concentration, phycocyanin, or dissolved oxygen; Pace et al. 2017; Butitta et al. 2017). For example, time series from automated sensors anticipated a cyanobacterial bloom in a whole-lake experiment (Pace et al. 2017). However, in several studies, state variables did not reflect EWI trends or they failed to respond concurrently (Burthe et al. 2016; Gsell et al. 2016; Spears et al. 2017; Rohde et al. 2022). Numerous i nteracting drivers (light, temperature, nutrients, etc.) can affect cyanobacterial bloom development, so it is likely that varying environmental conditions damper early warning i ndicators that could signal cyanobacterial blooms (Wilkinson et al. 2021). Buelo et al. (2022) expressed the need for system-specific examples to quantify the likelihood of regime shifts (from a clear-water to a cyanobacterial bloom state, for example). No substitutes exist for site-specific knowledge and experiments (Dakos et al. 2015). Often, indicators are only detected i n retrospect or while state transitions are occurring, or ecosystems have such a narrow range of variability that changes in EWIs might not be useful for predictive purpose (Van Nes & Scheffer 2007).Even with the variable success of using early warning indicators to predict ecosystem state changes in complex limnological conditions, there is consensus about the value of making estimates and analyzing trends for determining baseline indicator values (Dakos et al. 2015). A deviating trend from baseline values could forecast a transition in ecosystem state (Bestelmeyer et al. 2011; Peters et al. 2013; Wilkinson et al. 2018), although trends could still be noisy and difficult to interpret, and sometimes too unclear to initiate management action (Dakos et al. 2015). For example, managers can estimate how close a system is to a threshold based on ecosystem variable baselines, and then rank the vulnerability of systems at any given time to make management decisions. Thresholds for concern and/or action for cyanobacterial blooms can be created based on ecosystem variables like chlorophyll a, phycocyanin, and dissolved oxygen (Pace et al. 2017), and “resilience maps,” or estimates of resilience, can be used to prioritize management of different systems, although even in best-case scenarios, surprises in ecosystem state can occur with no warning (Dakos et al. 2015). High-frequency water quality monitoring and longer-term sampling will improve managers’ confidence in baseline data and subsequent management decisions. To be able to support decision-making, resilience indicators must be assessed in the context of ecological understanding of individual systems (Rohde et al. 2022).
Implications for HABs Vulnerability
Water quality conditions are difficult to forecast due to variability of water quality metrics outside baseline conditions, which puts tremendous strain on resource managers (Brookes et al. 2014; Khan et al. 2015; Carey et al. 2022). Regime shifts can occur with an abrupt transition from a clear-water state to an algal-dominated state (Scheffer et al. 2009) and impending regime shifts may sometimes be detected by frequent monitoring of water quality variables that could represent a shift to algal dominance, like chlorophyll a , phycocyanin, and dissolved oxygen saturation (Batt et al. 2013; Sirota et al. 2013; Ortiz et al. 2020). With predictions of increased water column stability with lake warming (Woolway et al. 2020), and of increased frequency and severity of storms, physical lake conditions may shift toward supporting cyanobacterial growth across a gradient of lake trophic states (Reinl et al. 2021). The resilience of similar systems may be ranked by estimating resilience indicators of the systems (Scheffer et al. 2015). Resilience as an indicator is relative and qualitative as opposed to absolute and quantitative because the dynamics of systems are complex and it is difficult to account for seasonal trends in time series of individual systems. Long-term monitoring provides baseline information about the stability of i ndividual systems and can be used to discern whether a system is losing or gaining resilience (Scheffer et al. 2015).Risk Assessment Scorecard
The risk assessment scorecard (Figure 21) supports our predictions based on water quality sampling that lakes like Jordan Pond have the fewest risk factors to future HAB development, while lakes like Witch Hole Pond have more risk factors and may be more vulnerable to developing HABs in the future.Lakes like Jordan Pond
(Long Pond, Eagle Lake, Bubble Pond, Upper Breakneck Pond)Oligotrophic lakes may be more resilient to processes like internal P loading that could trigger cyanobacterial blooms in other lakes (Orihel et al. 2017), and this heightened resilience may improve the chances of detecting an impending regime shift before it occurs (Ortiz et al. 2020). As we observed in phycocyanin data from 2020, surface aggregations and metalimnetic maxima of cyanobacteria can occur in oligotrophic lakes (Lofton et al. 2020), and although cyanobacteria in oligotrophic lakes may have low average biomass, even ephemeral surface blooms could present concerns for recreation or taste and odor of drinking water (Reinl et al. 2021). Deep l akes (>25 m) like Jordan Pond might be more resilient to anoxic conditions (Deeds et al. 2021), which is especially important with a declining proportion of oligotrophic lakes across the landscape (Stoddard et al. 2016). According to Wagner & Adrian (2009), certain water quality thresholds, including stable stratification that lasts longer than three weeks and a TP range between 70 to 125 µg L - 1, must be crossed for cyanobacterial bloom formers like Aphanizomenon, Dolichospermum, and Microcystis to dominate. While Jordan Pond and many other lakes in Acadia National Park stratify stably for >3 weeks each summer, none of the Northeast Temperate Network (NETN) lakes in ANP meet the TP criteria (Gawley & Wiggin 2016). Average Secchi depth in Jordan Pond has shallowed slightly since 1995, with a more notable shallowing trend since 2011. DOC has increased slightly, likely as a result of the successful Clean Air Act Amendments of 1990 and subsequent effects on lake-landscape l inkages. Nutrients, particularly TN, have decreased in Jordan Pond since 1995, while chlorophyll a has generally remained unchanged. These long-term data suggest that while Jordan Pond may be more at risk due to climate factors, Jordan Pond and similar ANP lakes l ikely have high resilience to cyanobacterial blooms and low risk for HABs.
However, in the last decade, Gloeotrichia echinulata, a type of colonial cyanobacteria that produces the toxin microcystin-LR, has been increasing in low-nutrient Maine lakes. The reasons for Gloeotrichia increases in low-nutrient lakes are unclear (Carey et al. 2012b), so it is important to be aware that Gloeotrichia may become problematic in lakes like Jordan Pond that have few risk factors for other HAB-forming cyanobacteria.
Lakes like Seal Cove Pond
(Echo Lake, Lake Wood, Upper Hadlock Pond, Lower Hadlock Pond, Lower Breakneck Pond, Aunt Betty Pond, Bear Brook Pond)Historical data show a declining trend in TP and TN in Seal Cove Pond since 1995, with a relatively stable TN:TP ratio (Figure 16). DOC, chl a, and Secchi have also maintained relatively stable averages since 1995. As an oligo-mesotrophic lake that stratifies, of moderate depth and flushing rate, Seal Cove Pond and similar lakes are likely at moderate risk of potential future HAB development. Three of the lakes (Lake Wood, Aunt Betty Pond, and Bear Brook Pond) in this moderate risk category do not stratify and have relatively fast flushing rates, so even though they are shallow and meso-eutrophic, we place them in the same risk category as Seal Cove Pond.
Lakes like Witch Hole Pond
(Round Pond, Hodgdon Pond, Seawall Pond)In shallow lakes that stratify (e.g. ≤8 m in Wagner & Adrian 2009), cyanobacteria may have easier access to the high nutrient concentrations in the hypolimnion (Carey et al. 2012a). Lakes in this category of considerable risk are shallow, meso-eutrophic, may or may not stratify, and have moderate flushing rates. They are likely more prone to and less resilient to internal nutrient l oading as a result of anoxic conditions. Since 1995, Secchi depth, chl a concentration, and TN:TP have remained relatively stable in Witch Hole Pond, while DOC, TN, and TP have declined. If regular monitoring of Witch Hole Pond revealed that Secchi depth, chl a, and/or TP surpassed the historical 75% quantile values (2.8 m, 4.7 µg L - 1, 11 µg L - 1, respectively; Table 2), resource managers might consider that Witch Hole Pond could be losing resilience, which could precede a switch to an alternative state, such as HAB development (Scheffer et al. 2001). In response to a driver like nutrient loading, shallow lakes may exhibit pronounced hysteresis, while deeper lakes often have greater resilience and respond more smoothly (Carpenter et al.1999). In eutrophic systems, abrupt shifts (also referred to as critical transitions) can occur in response to small changes in the system (Carpenter et al. 1999; Batt et al. 2013; Cottingham et al. 2015; Buelo et al. 2018).
While the widespread intensification of algal blooms in response to the effects of climate change and eutrophication has been hypothesized (Elliott 2012, O’Neil et al. 2012, Chapra et al. 2017), other evidence shows different patterns (Kraemer et al. 2017; Wilkinson et al. 2021). Oliver et al. (2017) did not find a widespread increase in chlorophyll a and nutrient concentration in a large study of lakes in the Northeast United States, and Wilkinson et al. (2021) showed that smaller lakes are more likely to have negative trends in algal bloom magnitude and severity, especially those that benefited from restoration and protection by the Clean Water Act of 1972. Following these findings, lakes within ANP, even those we have placed in the category of considerable risk, may have an extra layer of protection from HAB formation and development as a result of the long-term water quality monitoring by ANP resource managers.
Recommendations
Management Options
Due to a long evolutionary history, the adaptability and tolerance of blooming cyanobacterial taxa to short-term (diel, seasonal, decadal) and long-term (geological) environmental pressures l ike climate change make cyanobacteria a “group for all seasons” (Paerl 2001). The most successful management strategies will likely incorporate multi-level responses to potential future HABs (Watson et al. 2015), as ANP manages for change, not just for persistence (Schuurman ANP Science Symposium 2021).Within the Resist-Accept-Direct decision framework (Fisichelli et al. 2016b; Schuurman et al. 2020), a range of management options exist for managers of lakes that may experience or are currently experiencing HABs. In cases where it is decided that no action will be taken to shape the trajectory of change, the decision to “Accept” is selected. If management action will be taken to preserve a historical condition, the management decision is to “Resist.” The decision to “Direct” is made when the desired outcome is to create a new set of conditions (Schuurman et al. 2020). Our goal is to identify possible management approaches within the RAD framework based on lake vulnerability to HABs, and to present these recommendations clearly. Selection ofmanagement approaches will depend on the NPS mission, goals of ANP resource managers, stakeholder preferences, societal values, and specific desired outcomes. The best approaches will be manager-centered, simple, and intuitive (Schuurman 2021).
For lakes with some level of vulnerability to HABs or that are of special importance to resource managers and stakeholders, but are not currently experiencing HAB conditions, the “Accept” decision framework could include targeted monitoring programs and public communication and outreach. For lakes with active HABs, the “Resist” approach could incorporate management strategies like external nutrient control, algaecide application, internal nutrient manipulation, de-stratification through mixing or aeration, or biological control (Watson et al. 2015). Ideally, the outcomes of decision-making approaches will be shared collaboratively to support management decisions as issues with freshwater HABs arise across NPS units. For example, in recent years Isle Royale National Park has posted advisories warning visitors not to drink or recreate in lakes affected by HABs, along with educational descriptions of HAB appearance, causes, and impacts on humans, animals, and the ecosystem. This form of visitor communication is an example of an “Accept” approach. It aims to protect the health and safety of visitors, but does not reduce the magnitude of HABs or restore lakes to historical, pre-HAB conditions
Resist
Returning lakes to pre-HAB conditions, when possible, can be burdensome and expensive, and resulting impacts on other aspects of the ecosystem may be difficult to predict or control. HAB and non-HAB assemblages often include overlapping populations of various growth stages that are regulated by factors with spatial and temporal heterogeneity (e.g. light, nutrients, seeding, cell division, grazing; Watson et al. 2015). Management decisions based on measures of biovolume, chlorophyll a or other pigments, or cell counts may be inefficient because these metrics can vary widely across time and space and may be uncoupled from HAB conditions (Harris 1980; Watson et al. 2015). However, several management strategies exist as short-term responses for reducing exposure to and minimizing health impacts of HABs (Watson et al. 2015). While the best long-term management approach is prevention of HABs (Paerl et al. 2001), management approaches are also available for sustained reduction of HAB frequency and severity (Watson et al. 2015).A long-term approach is to reduce external nutrient loads through watershed and in-lake nutrient management. This approach is the most practical and cost-effective strategy for reducing HABs (Paerl et al. 2001; Watson et al. 2015; Buelo et al. 2018). Examples of this approach include reductions of detergent- and sewage-based P inputs. Most of the lakes in ANP are fully protected from these sorts of nutrient inputs. Other sources of nutrient inputs could include shoreline erosion, which may vary with trail usage near the lake and within the watershed, and type and amount of vegetation in the watershed. In ANP, climate change effects like increasing temperature and increasing frequency and intensity of storms are impacting the habitat suitability for many tree species and thus forest composition (Fisichelli et al. 2013). These shifts i n forest composition will have implications for the timing and magnitude of pulses of nutrients transferred from the landscape to lakes. HAB magnitude may be tied to the severity of extreme precipitation events, particularly in eutrophic lakes (Wilkinson et al. 2021). In these cases, resource managers may want to consider strategies for minimizing nutrient inputs from the watershed and methods for reducing storm-induced internal loading via sediment resuspension (Wilkinson et al. 2021). However, most external nutrient inputs come from nonpoint sources, and are difficult or impossible for resource managers to control (Pace et al. 2017). The safest management approach for freshwater HABs is to protect the resilience of desirable conditions by regulating external nutrient loads to lakes and maintaining a “safe operating space” for lake processes (Pace et al. 2017).
Other approaches to control freshwater HABs vary by lake type and desired outcomes, or if the l ake is a source of drinking water. The depth at which drinking water is extracted may be altered (Carey et al. 2022), or toxin removal from drinking water may be necessary (Dugan et al. 2018). Algaecides like copper sulfate (Paerl et al. 2001) and hydrogen peroxide (Reinl et al. 2021) have been used to target nuisance algae (Buelo et al. 2018). In addition, magnetic coagulants (Liu et al. 2013) and ultrasound (Wu et al. 2011) have been used to influence algal community composition and inhibit cyanobacterial growth, respectively (Pace et al. 2017; Reinl et al. 2021). Depending on internal nutrient conditions and the sensitivity of a lake to developing naturally-occurring anoxia, artificial aeration of the hypolimnion or chemical treatments for the precipitation of P from the water column may be suitable management approaches (Deeds et al. 2021). Vertical destratification through mechanical mixing or aeration can reduce the competitive advantage of cyanobacteria for light and nutrients (Paerl et al. 2001; Visser et al. 2016). Enhanced water flushing to reduce retention time, while costly, may be plausible in systems with abundant upstream reservoirs (Paerl et al. 2001; Watson et al. 2015; Paerl et al. 2018).
Accept
Monitoring Approaches
The Air & Water Program at ANP has a decades-long legacy of air and water quality monitoring. Long-term continuous monitoring is essential for understanding ecosystem processes and detection of early warning indicators of ecosystem change (Dakos et al. 2015; Burthe et al. 2016), like HAB development. Highly-resolved temporal and spatial data are most useful for making informed and effective management decisions (Rohde et al. 2022), particularly for drinking water sources (Wilkinson et al. 2018), but the monitoring burden required to correlate HABs with specific environmental drivers may be implausible (Ho & Michalak 2020). It is i mportant to balance sampling frequency across lakes to capture potential HAB dynamics with realistic expectations of ANP staff and resource allocation (e.g. Wilkinson et al. 2021). Based on the ranking of lakes on the Risk Assessment Scorecard, we suggest integrating sustainable, easily repeatable, and easy-to-implement steps into the current Air & Water monitoring program. We recommend that resource managers direct monitoring efforts to shallow meso- and eutrophic lakes like Seal Cove Pond and Witch Hole Pond, particularly during mid- to late-summer when water temperature is high and dissolved oxygen is low, and into the fall, when high wind speeds accelerate the breakdown of thermal stratification and internal nutrients from sediments could be mixed into the water column. Additionally, as Gloeotrichia is increasing i n oligotrophic lakes across Maine, we recommend routine monitoring for Gloeotrichia colonies in Jordan Pond (and other oligotrophic lakes if possible) beginning in early June.Another water quality parameter to examine more closely in routine monitoring is phycocyanin fluorescence. Phycocyanin is a photosynthetic cyanobacterial pigment. Phycocyanin fluorescence gives a relative estimate of cyanobacterial abundance, and can be measured by the total algae sensor on the EXO used in current monitoring protocols. Sometimes calibration of the total algae sensor can present problems with data accuracy. If calibration of the total algae sensor is a concern, a handheld fluorometer may be purchased relatively inexpensively, and can be used temporarily or on a longer-term basis to compare surface water phycocyanin trends with measurements from the EXO sensor. Recording monthly phycocyanin measurements throughout the water column of ANP lakes will be important for developing baselines for each lake. After a few seasons of regular phycocyanin measurements, data points that fall outside the 75 t h percentile may trigger additional sampling.
High frequency (hourly to daily) phycocyanin monitoring would capture periods of high risk that are missed by monthly sampling (McQuaid et al. 2011), particularly after extreme precipitation events that may increase nutrient loading from the landscape (Heisler et al. 2008).
High-frequency phycocyanin sensor data, similar to that recorded by sensors attached to the Jordan Pond Buoy, would help managers decide when/if to perform cyanotoxin sampling, issue public advisories, and/or alter drinking water intakes (McQuaid et al. 2011; Wong & Hobbs 2020). High-frequency monitoring equipment can be costly and requires extra time and effort from staff for annual set-up, take-down, maintenance, and troubleshooting, and so decision-makers would need to consider on which lake(s) and on what timeline the installation of high-frequency monitoring equipment would be most informative. When selecting lakes, we recommend prioritizing drinking water sources, lakes of great recreational value, and/or lakes ranked at “considerable” risk for HAB development. Inferences about phycocyanin dynamics may be made about un-instrumented lakes with similar water quality and morphometric characteristics.
In addition to increasing the temporal resolution of sampling, increasing the spatial resolution of sampling would capture more of the spatial heterogeneity of water quality parameters in lakes (Duggan et al. 2018; Woolway et al. 2020). Spatial heterogeneity is common in lakes, and i nstead of sampling only the deepest point in the lake, broader spatial sampling would improve our understanding of lake-wide algal dynamics and ability to identify and detect early warning i ndicators of HABs (Rohde et al. 2022). One relatively inexpensive method of increasing spatial resolution of sampling is increasing the number of temperature and DO strings deployed in l akes. Pace et al. (2017) recommend collecting samples not only in the deep holes of lakes, but also near areas closer to shore or in expected algal hotspots (Molot et al. 2014; Ortiz et al. 2020). For example, the northern and eastern edges of Jordan Pond, both basins of Seal Cove Pond, and Witch Hole Pond would be desirable areas for sampling, as these areas may be more vulnerable to HAB development.
Another method for increasing spatial and temporal data collection is to broaden winter sampling of ANP lakes. For example, ice phenology (ice-on and ice-out dates, and the interval and ice dynamics between), which impacts many aspects of algal community structure, could be recorded with on-site time-lapse cameras for select lakes (Couture et al. 2015; Carey et al. 2022). In addition, continuous under-ice sensor monitoring would provide data on spring phytoplankton blooms that are often missed with traditional sampling methods (Hampton et al. 2017; Ortiz et al. 2020).
A multi-pronged approach to data collection (i.e. high-frequency in-situ buoy measurements, l ong-term water column sampling, nutrient limitation experiments, and paleolimnology) has been valuable for understanding ecological dynamics in Jordan Pond and other ANP lakes (e.g. Daggett et al. 2015; Strock et al. 2017; Malik et al. 2018; Warner et al. 2018; Fowler et al. 2022). Collecting sediment samples for eDNA analysis would provide key information about which cyanobacterial taxa are present in each lake and would complement the risk assessment scorecard developed in this project. Because cyanobacterial taxa respond differently to effects of climate resulting in changes to stratification and nutrient loading (Carey et al. 2012a), better understanding which taxa are present in ANP lakes will improve HAB forecasting.
Although identifying early warning indicators of HABs is easier to do post hoc than in real-time (Pace et al. 2017), it may be beneficial to lake managers to develop a system for receiving alerts when monthly data points for key water quality variables (e.g. Secchi, temperature, DO, phycocyanin, chl a ) exceed the 75 t h percentile. This alert would trigger an examination of the data to verify the exceedance, followed by a decision-making process for when/if to re-sample, publish an advisory for ANP visitors, collect water samples for cyanobacterial identification and/or toxin analysis, and/or redirect water intakes away from surface or metalimnetic blooms (Reinl et al. 2021). Assuming increases in early warning indicators appear days or weeks before a critical transition (Ortiz et al. 2020), these alerts could give lake managers time to decide upon a course of action (Wilkinson et al. 2018). Seasonal or annual comparisons of key water quality parameters for each lake paired with a summary report illustrating trends will inform education and outreach efforts.
Communication, Education, & Outreach
Air & Water Quality Program scientists at ANP began contributing to the nationwide Cyanobacteria Monitoring Collaborative programs bloomWatch and Cyanoscope, and have since initiated SPATT sampling (a form of cyanotoxin tracking) and quantitative PCR (qPCR) in Jordan Pond, Lake Wood, and Upper Hadlock Pond. In the past decade, Jordan Pond Buoy outreach projects like the interactive kiosk in the Jordan Pond House and social media communications have inspired curiosity about ANP lakes in thousands of in-person and virtual visitors worldwide. Expanding citizen science across ANP will amplify visitors’ awareness of ANP lake science. A display about HABs at the Sieur de Monts Nature Center Spring, for example, could spur visitors’ interest in freshwater algal dynamics at ANP.Across New England, several states have developed HABs advisories based on water transparency, cell counts, pigments, and/or taxonomy (Bacon 2016). ANP managers can learn from and adapt these states’ protocols to prepare for, measure, track, and respond to potential HAB conditions.
For example, the New Hampshire Department of Environmental Services (NHDES) maintains a “Lake Information Mapper” GIS tool that allows users to view cyanobacterial bloom history of i ndividual lakes. The NHDES Cyanobacterial HAB Program issues both advisories and alerts for individual waterbodies. An advisory report is issued when cyanobacteria cell concentrations reach >70,000 cells ml - 1 or >50% of a sample is cyanobacteria (NHDES 2022). Advisories may be issued between May 15 and October 15, and resampling is performed weekly until the bloom subsides. Alerts can be issued on the basis of a photograph before water samples can be analyzed, or if a water sample has cyanobacterial cell concentrations approaching >70,000 cells ml - 1 . Alerts, which may be issued year-round as needed, are valid for one week, and may turn i nto advisories or may expire if the bloom passes (NHDES 2022). Cyanobacterial HAB advisories and alerts are updated daily on the Healthy Swimming Mapper, an integrative tool that allows users to track alerts and advisories spatially and temporally. Similarly, the Vermont Department of Health and Department of Environmental Conservation
(DEC) developed the “Vermont Cyanobacteria Tracker,” a GIS tool that gives the most current cyanobacterial reports for a given lake, along with photos of the bloom and a table with that l ake’s bloom history for the current year (Vermont Department of Health 2023).
Within ANP, communicating with visitors about risk management and resource closures will be i mportant for protecting visitor safety and minimizing future HAB-related illness or injury. These forms of communication could take the form of online alerts and virtual notifications through nps.gov and posted signage at affected lakes. As various stakeholders access ANP lakes for various uses, these communications should include risk levels and recommendations for a variety of resource uses. For example, is the risk of skin irritation from cyanotoxins high enough that swimmers should be directed to another area? Should anglers avoid skin contact with the water?
Communication and collaboration with community organizations like local homeowner and conservation groups will be important for communicating risks and recommendations to the public and motivating public awareness and support for lake protection from future HABs. Identifying potential stakeholders to include in a “HABs awareness and support” network and then providing regular summaries of water quality monitoring and implications for public use will advance ANP outreach and education efforts. For example, homeowners on Hodgdon Pond have voiced concern about water level fluctuations due to beaver activity and culverts. As Hodgdon Pond is also at considerable risk of future HABs, these homeowners would ideally be i ncluded in conversations about HABs risks, in which they might also share their own long- or short-term observations and knowledge about ecological changes in Hodgdon Pond. Similar conversations with homeowners and anglers on Seal Cove Pond, a popular sport fishing lake at moderate risk of future HABs, could provide valuable information for ANP resource managers and a variety of stakeholders. Maine Coast Heritage Trust (MCHT) supports a range of i nitiatives for increasing resilience of coastal Maine resources to climate change and works to foster connections among communities, groups, and individuals interested in preserving and protecting these resources. While MCHT is a land conservation organization, its ongoing support of outdoor recreation, watershed protection and water access, and experience with community outreach could make it a valuable local partner for advancing awareness among shared stakeholders.
Limitations & Future Work
A strength of this project is its multi-faceted approach to data collection, including historical water and air quality records, long-term continuous buoy data, and a season of targeted water quality sampling to address questions specific to this project. The targeted water quality sampling during the spring, summer, and fall of 2020 illuminated important information about water quality metrics in lakes of different trophic states to better understand their vulnerability to HABs. This sort of targeted sampling, including continuous temperature and dissolved oxygen sensor measurements and biweekly water column measurements of chlorophyll a , phycocyanin, and nutrients, would be even more useful if expanded to subsequent years and sample sites. For example, a 5- or 10-year dataset including more lakes of varying morphometry, flushing rates, and trophic states would allow us to further tailor risk assessments to specific lakes in ANP. For the purpose of delivering realistic recommendations for management planning, we have tried to balance precision across ANP lakes with generalized assumptions about lake types in ANP based on trophic status and level of protection.
Due to the challenges of launching a research project at the beginning of 2020, measurements of phycocyanin and chlorophyll a immediately after i ce-off are missing from the Witch Hole Pond dataset. We expect that, similar to Jordan Pond and Seal Cove Pond, there was elevated phycocyanin in surface waters at this time, but it would be useful to collect data immediately after ice-off to support these assumptions. We are also missing phytoplankton cell density counts from Witch Hole Pond for the 2020 season, which would help us understand whether HAB-forming cyanobacterial taxa were present in Witch Hole Pond in 2020. Regardless, this gap in data does not change our conclusions about the vulnerability of Witch Hole Pond to future HABs.
Winter water quality monitoring would also help fill a gap in understanding about the likelihood of HAB formation and persistence. The Global Lake Ecological Observatory Network (GLEON) and others have recently shown the importance of snow and ice dynamics on dissolved oxygen, l ight availability, thermal structure, phytoplankton community structure, and nutrient levels throughout the winter and in subsequent seasons (Beall et al. 2016; Hrycik & Stockwell 2021; Jansen et al. 2021; Hrycik et al. 2022). Winter monitoring is of the highest importance considering the dramatic changes in snow and ice cover on temperate and boreal lakes (Ozersky et al. 2021), particularly in ANP and throughout Maine (Beyene & Jain 2015; Boeff et al. 2016; Warner et al. 2018).
More comprehensive identification of cyanobacterial taxa would be useful in future projects. With the exception of Dolichospermum i n Seal Cove Pond during a spring sampling event, the lakes in this project were largely absent of toxin-producing cyanobacterial taxa. However, Microcystis may have been too small to identify microscopically. Environmental DNA (eDNA) and sediment DNA (sedDNA) will be important tools for identifying which toxin-producing cyanobacteria taxa are present in water samples and putting these results in the context of historical change. The University of Maine has a Water Resources Research Institute (WRRI)-funded eDNA project for investigating how warming winters over the past 125 years
have impacted HAB-forming cyanobacteria in Maine lakes. eDNA has already been used to identify Dolichospermum as one of the toxin-producing taxa in an August 2020 HAB event in Damariscotta Lake (Bartow 2023).
Furthermore, in this study we had no measure for whether phycocyanin concentration was correlated with toxicity. It is difficult to predict if and when an algal bloom will become harmful, as not all toxin-producing taxa produce toxins in all conditions, and the amounts of toxins may vary with light, temperature, and nutrients (Otten & Paerl 2015; Wurtsbaugh et al. 2019). A lack of l ong-term cyanobacterial bloom data restricts our interpretations of the limited data researchers have collected (Ho & Michalak 2017). Contributions to the Cyanobacteria Monitoring Collaborative programs bloomWatch and Cyanoscope, as well as SPATT sampling (a form of toxin tracking) and qPCR analysis in select lakes will improve our understanding of conditions precluding potential future HABs in ANP lakes. If concerns arise within particular lakes, water samples could be sent to labs for testing of specific cyanobacterial toxins. The Maine DEP has been testing water from lakes in Maine for microcystins since 2008.
Acknowledgements
This work is funded by a Second Century Stewardship award from the Schoodic Institute at Acadia National Park. We are grateful to Václava Hazuková, Katie Paul, Joe Mohan, and Ben Burpee for assistance in the field, as well as project consulting from Linda Bacon and Jeremy Deeds from the Maine Department of Environmental Protection and Charlie Culbertson from the U.S. Geological Survey. Kathleen Brown and Jake Van Gorder from the NPS and Kate Warner from the Maine Rural Water Association contributed valuable comments to the manuscript.The findings and conclusions in this article are those of the authors and do not necessarily represent the views of the U.S. Department of Interior or U.S. Government.
Adrian, R., O'Reilly, C. M., Zagarese, H., Baines, S. B., Hessen, D. O., Keller, W., Livingstone, D. M., Sommaruga, R., Straile, D., Van Donk, E., Weyhenmeyer, G. A., & Winder, M. (2009). Lakes as sentinels of climate change. Limnology and Oceanography, 54 (6part2), 2283-2297.
Agbeti, M. D., & Smol, J. P. (1995). Winter limnology: a comparison of physical, chemical and biological characteristics in two temperate lakes during ice cover. Hydrobiologia, 304 , 221-234.
Alin, S. R., & Johnson, T. C. (2007). Carbon cycling in large lakes of the world: A synthesis of production
American Public Health Association. (2000). Standard Methods for the Examination of Water and Wastewater (20 th Ed.). [Eaton, A., et al. (eds.)]. Washington, D. C., American Public Health Association Press
Amirbahman, A., Norton, S., Fitzgibbon, K., Pavri, F., Bacon, L., & Williams, S. (2016). Maine Lakes: Living on the Edge? [PowerPoint slides]. University of Maine Mitchell Center for Sustainability Solutions Seminar.
Anneville, O., Domaizon, I., Kerimoglu, O., Rimet, F., & Jacquet, S. (2015). Blue-green algae in a “Greenhouse Century”? New insights from field data on climate change impacts on cyanobacteria abundance. Ecosystems, 18 (3), 441-458.
Arheimer, B., Andréasson, J., Fogelberg, S., Johnsson, H., Pers, C. B., & Persson, K. (2005). Climate change impact on water quality: Model results from southern Sweden. AMBIO: A Journal of the Human Environment, 34 (7), 559-566.
Aubriot, L., & Bonilla, S. (2012). Rapid regulation of phosphate uptake in freshwater cyanobacterial blooms. Aquatic Microbial Ecology, 67 (3), 251-263.
Austin, J. A., & Colman, S. M. (2007). Lake Superior summer water temperatures are increasing more rapidly than regional air temperatures: A positive ice ‐ albedo feedback. Geophysical Research Letters, 34 (6).
Bacon, L. (2016). Harmful Algal Blooms and Cyanotoxins in Maine [PowerPoint slides]. Volunteer Lake Monitoring Program Annual Meeting.
Bartow, E. (2023, July 20). Investigating Harmful Algae Blooms with Robin Sleith. Maine EPSCoR. https://umaine.edu/epscor/2023/07/20/investigating-harmful-algae-blooms-with-robin-sleith/
Batt, R. D., Carpenter, S. R., Cole, J. J., Pace, M. L., & Johnson, R. A. (2013). Changes in ecosystem resilience detected in automated measures of ecosystem metabolism during a whole-lake manipulation. Proceedings of the National Academy of Sciences , 110 (43), 17398-17403.
Beall, B. F. N., Twiss, M. R., Smith, D. E., Oyserman, B. O., Rozmarynowycz, M. J., Binding, C. E., Bourbonniere, R. A., Bullerjahn, G. S., Palmer, M. E., Reavie, E. D., Waters, M. K., Woityra, W. C., & McKay, R. M. L. (2016). Ice cover extent drives phytoplankton and bacterial community structure in a l arge north ‐ temperate lake: implications for a warming climate. Environmental Microbiology , 18 (6), 1704-1719.
Beaulieu, M., Pick, F., Palmer, M., Watson, S., Winter, J., Zurawell, R., & Gregory-Eaves, I. (2014). Comparing predictive cyanobacterial models from temperate regions. Canadian Journal of Fisheries and Aquatic Sciences , 71 (12), 1830-1839.
Bestelmeyer, B. T., Ellison, A. M., Fraser, W. R., Gorman, K. B., Holbrook, S. J., Laney, C. M., Ohman, M. D., Peters, D. P. C., Pillsbury, F. C., Rassweiler, A., Schmitt, R. J., & Sharma, S. (2011). Analysis of abrupt transitions in ecological systems. Ecosphere, 2 (12), 1-26.
Beyene, M. T., & Jain, S. (2015). Wintertime weather ‐ climate variability and its links to early spring ice ‐ out i n Maine lakes. Limnology and Oceanography , 60 (6), 1890-1905.
Boeff, K. A., Strock, K. E., & Saros, J. E. (2016). Evaluating planktonic diatom response to climate change across three lakes with differing morphometry. Journal of Paleolimnology , 56 , 33-47.
Blumberg, A. F., & Di Toro, D. M. (1990). Effects of climate warming on dissolved oxygen concentrations i n Lake Erie. Transactions of the American Fisheries Society , 119 (2), 210-223.
Brookes, J. D., Carey, C. C., Hamilton, D. P., Ho, L., van der Linden, L., Renner, R., & Rigosi, A. (2014). Emerging challenges for the drinking water industry. Environmental Science and Technology, 48 (4), 2099-2101.
Brookes, J. D., & Carey, C. C. (2011). Resilience to blooms. Science , 334 (6052), 46-47.
Brothers, S., Köhler, J., Attermeyer, K., Grossart, H. P., Mehner, T., Meyer, N., Scharnweber, K., & Hilt, S. (2014). A feedback loop links brownification and anoxia in a temperate, shallow lake. Limnology and Oceanography , 59 (4), 1388-1398.
Brunberg, A. K., & Blomqvist, P. (2002). Benthic overwintering of Microcystis colonies under different environmental conditions. Journal of Plankton Research , 24 (11), 1247-1252.
Buelo, C. D., Carpenter, S. R., & Pace, M. L. (2018). A modeling analysis of spatial statistical indicators of thresholds for algal blooms. Limnology and Oceanography Letters , 3 (5), 384-392.
Buelo, C. D., Pace, M. L., Carpenter, S. R., Stanley, E. H., Ortiz, D. A., & Ha, D. T. (2022). Evaluating the performance of temporal and spatial early warning statistics of algal blooms. Ecological Applications , 32 (5), e2616.
Burthe, S. J., Henrys, P. A., Mackay, E. B., Spears, B. M., Campbell, R., Carvalho, L., Dudley, B., Gunn, I. D. M., Johns, D. G., Maberly, S. C., May, L., Newell, M. A., Wanless, S., Winfield, I. J., Thackeray, S. J., & Daunt, F. (2016). Do early warning indicators consistently predict nonlinear change in long ‐ term ecological data?. Journal of Applied Ecology , 53 (3), 666-676.
Butitta, V. L., Carpenter, S. R., Loken, L. C., Pace, M. L., & Stanley, E. H. (2017). Spatial early warning signals in a lake manipulation. Ecosphere , 8 (10), e01941.
Cao, H. S., Kong, F. X., Luo, L. C., Shi, X. L., Yang, Z., Zhang, X. F., & Tao, Y. (2006). Effects of wind and wind-induced waves on vertical phytoplankton distribution and surface blooms of Microcystis aeruginosa i n Lake Taihu. Journal of Freshwater Ecology , 21 (2), 231-238.
Carlson, R. E., & Simpson, J. (1996). A biomass based trophic state index. North American Lake Management Society .
Carey, C. C., Ewing, H. A., Cottingham, K. L., Weathers, K. C., Thomas, R. Q., & Haney, J. F. (2012b). Occurrence and toxicity of the cyanobacterium Gloeotrichia echinulata in low-nutrient lakes in the northeastern United States. Aquatic Ecology , 46 , 395-409.
Carey, C. C., Ibelings, B. W., Hoffmann, E. P., Hamilton, D. P., & Brookes, J. D. (2012a). Eco-physiological adaptations that favour freshwater cyanobacteria in a changing climate. Water Research , 46 (5), 1394-1407.
Carey, C. C., Woelmer, W. M., Lofton, M. E., Figueiredo, R. J., Bookout, B. J., Corrigan, R. S., Daneshmand, V., Hounshell, A. G., Howard, D. W., Lewis, A. S. L., McClure, R. P., Wander, H. L., Ward, N. K., & Thomas, R. Q. (2022). Advancing lake and reservoir water quality management with near-term, i terative ecological forecasting. Inland Waters , 12 (1), 107-120.
Carpenter, S. R., Ludwig, D., & Brock, W. A. (1999). Management of eutrophication for lakes subject to potentially irreversible change. Ecological Applications , 9 (3), 751-771.
Wilkinson, G. M., Carpenter, S. R., Cole, J. J., Pace, M. L., Batt, R. D., Buelo, C. D., & Kurtzweil, J. T. (2018). Early warning signals precede cyanobacterial blooms in multiple whole ‐l ake experiments. Ecological Monographs , 88 (2), 188-203.
Cavaliere, E., Fournier, I. B., Hazuková, V., Rue, G. P., Sadro, S., Berger, S. A., Cotner, J. B., Dugan, H. A., Hampton, S. E., Lottig, N. R., McMeans, B. C., Ozersky, T., Powers, S. M., Rautio, M., & O'Reilly, C.
M. (2021). The lake ice continuum concept: influence of winter conditions on energy and ecosystem dynamics. Journal of Geophysical Research: Biogeosciences , 126 (11), e2020JG006165.
Center for Disease Control and Prevention. (2023). Harmful Algal Bloom (HAB)-Associated Illness. https://www.cdc.gov/habs/index.html
Chapra, S. C., Boehlert, B., Fant, C., Bierman Jr, V. J., Henderson, J., Mills, D., ... & Paerl, H. W. (2017). Climate change impacts on harmful algal blooms in US freshwaters: a screening-level assessment. Environmental Science & Technology , 51 (16), 8933-8943.
Chorus, I., & Welker, M. (Eds.). (2021). Toxic Cyanobacteria in Water: A Guide to Their Public Health Consequences, Monitoring and Management (2nd ed.). CRC Press. https://doi.org/10.1201/9781003081449
Clilverd, H., White, D., & Lilly, M. (2009). Chemical and Physical Controls on the Oxygen Regime of Ice ‐ Covered Arctic Lakes and Reservoirs 1. JAWRA Journal of the American Water Resources Association , 45 (2), 500-511.
Coles, J. F., & Jones, R. C. (2000). Effect of temperature on photosynthesis ‐l ight response and growth of four phytoplankton species isolated from a tidal freshwater river. Journal of Phycology , 36 (1), 7-16.
Cottingham, K. L., Ewing, H. A., Greer, M. L., Carey, C. C., & Weathers, K. C. (2015). Cyanobacteria as biological drivers of lake nitrogen and phosphorus cycling. Ecosphere , 6 (1), 1-19.
Couture, S., Houle, D., & Gagnon, C. (2012). Increases of dissolved organic carbon in temperate and boreal lakes in Quebec, Canada. Environmental Science and Pollution Research , 19 (2), 361-371.
Couture, R. M., de Wit, H. A., Tominaga, K., Kiuru, P., & Markelov, I. (2015). Oxygen dynamics in a boreal l ake responds to long ‐ term changes in climate, ice phenology, and DOC inputs. Journal of Geophysical Research: Biogeosciences , 120 (11), 2441-2456.
Creed, I. F., Bergström, A. K., Trick, C. G., Grimm, N. B., Hessen, D. O., Karlsson, J., Kidd, K. A., Kritzberg, E., McKnight, D. M., Freeman, E. C., Senar, O. E., Andersson, A., Ask, J., Berggren, M., Cherif, M., Giesler, R., Hotchkiss, E. R., Kortelainen, P., Palta, M. M., Vrede, T., & Weyhenmeyer, G. A. (2018). Global change ‐ driven effects on dissolved organic matter composition: Implications for food webs of northern lakes. Global Change Biology , 24 (8), 3692-3714.
Daggett, C. T., Saros, J. E., Lafrancois, B. M., Simon, K. S., & Amirbahman, A. (2015). Effects of i ncreased concentrations of inorganic nitrogen and dissolved organic matter on phytoplankton in boreal l akes with differing nutrient limitation patterns. Aquatic Sciences , 77 , 511-521.
Dakos, V., Carpenter, S. R., van Nes, E. H., & Scheffer, M. (2015). Resilience indicators: prospects and l imitations for early warnings of regime shifts. Philosophical Transactions of the Royal Society B: Biological Sciences , 370 (1659), 20130263.
Dawson, T. P., Jackson, S. T., House, J. I., Prentice, I. C., & Mace, G. M. (2011). Beyond predictions: biodiversity conservation in a changing climate. Science , 332 (6025), 53-58.
Deeds, J., Amirbahman, A., Norton, S. A., Suitor, D. G., & Bacon, L. C. (2021). Predicting anoxia in l ow ‐ nutrient temperate lakes. Ecological Applications , 31 (6), e02361.
Deng, J., Qin, B., Paerl, H. W., Zhang, Y., Ma, J., & Chen, Y. (2014). Earlier and warmer springs increase cyanobacterial (Microcystis spp.) blooms in subtropical Lake Taihu, China. Freshwater Biology , 59 (5), 1076-1085.
Downing, J. A., Watson, S. B., & McCauley, E. (2001). Predicting cyanobacteria dominance in lakes. Canadian Journal of Fisheries and Aquatic Sciences , 58 (10), 1905-1908.
Dugan, N. R., Smith, S. J., & Sanan, T. T. (2018). Impacts of potassium permanganate and powdered activated carbon on cyanotoxin release. Journal ‐ American Water Works Association , 110 (11), E31-E42.
Easterling, D. R., Kunkel, K. E., Arnold, J. R., Knutson, T., LeGrande, A. N., Leung, L. R., Vose, R. S., Waliser, D. E., & Wehner, M. F. (2017). Precipitation change in the United States. In: Climate Science Special Report: Fourth National Climate Assessment, Volume I [Wuebbles, D.J., Fahey, D. W., Hibbard, K. A., Dokken, D. J., Stewart, B. C., & Maycock, T. K. (eds.)]. U.S. Global Change Research Program, Washington, DC, USA, pp. 207-230, doi: 10.7930/J0H993CC.
Elliott, J. A., Jones, I. D., & Thackeray, S. J. (2006). Testing the sensitivity of phytoplankton communities to changes in water temperature and nutrient load, in a temperate lake. Hydrobiologia , 559 , 401-411.
Elliott, J. A. (2012). Is the future blue-green? A review of the current model predictions of how climate change could affect pelagic freshwater cyanobacteria. Water Research , 46 (5), 1364-1371.
Ellis, A. W., & Greene, T. R. (2019). Synoptic climate evidence of a late-twentieth century change to earlier spring ice-out on Maine Lakes, USA. Climatic Change , 153 (3), 323-339.
Elser, J. J. (1999). The pathway to noxious cyanobacteria blooms in lakes: the food web as the final turn. Freshwater Biology , 42 (3), 537-543.
Fee, E. J., Hecky, R. E., Kasian, S. E. M., & Cruikshank, D. R. (1996). Effects of lake size, water clarity, and climatic variability on mixing depths in Canadian Shield lakes. Limnology and Oceanography , 41 (5), 912-920.
Fernandez, I.J., Schmitt, C., Birkel, S., Stancioff, E., Pershing, A., Kelley, J., Runge, J., Jacobson, G., & Mayewski, P. (2015). Maine’s Climate Future: 2015 Update. Orono, ME: University of Maine. 24pp
Fernandez, I., Birkel, S., Schmitt, C., Simonson, J., Lyon, B., Pershing, A., Stancioff, E., Jacobson, G., & and Mayewski, P. (2020). Maine’s Climate Future 2020 Update. Orono, ME: University of Maine. climatechange.umaine.edu/climate-matters/maines-climate-future/
Finstad, A. G., Helland, I. P., Ugedal, O., Hesthagen, T., & Hessen, D. O. (2014). Unimodal response of fish yield to dissolved organic carbon. Ecology Letters , 17 (1), 36-43.
Fisichelli, N.A., Peters, M., Iverson, L., Matthews, S., & Hawkins Hoffman, C. (2013). Climate change and forests of the Acadia National Park region: Projected changes in habitat suitability for 83 tree species. Natural Resource Report NPS/ACAD/NRR—2013/733. National Park Service, Fort Collins, Colorado.
Fisichelli N. A., Schuurman, G. W., Symstad, A. J., Ray, A., Miller, B., Cross, M., & Rowland, E. (2016b). Resource Management and Operations in Southwest South Dakota: Climate Change Scenario Planning Workshop Summary January 20–21 2016, Rapid City, SD. National Park Service. Report no.
NPS/NRSS/NRR—2016/1289.
Freeman, E. C., Creed, I. F., Jones, B., & Bergström, A. K. (2020). Global changes may be promoting a rise in select cyanobacteria in nutrient ‐ poor northern l akes. Global Change Biology , 26 (9), 4966-4987.
Foley, B., Jones, I. D., Maberly, S. C., & Rippey, B. (2012). Long ‐ term changes in oxygen depletion i n a small temperate lake: effects of climate change and eutrophication. Freshwater Biology , 57 (2), 278-289.
Fowler, R. A., Warner, K. A., Gawley, W. G., & Saros, J. E. (2022). Paleolimnological comparison of algal changes in a clear-versus a brown-water lake over the last two centuries in the northeastern USA. Journal of Paleolimnology , 67 (3), 289-305.
Gawley, B. (2023). A Little Ice Would Be Nice. ACADIA Journal, Winter/Spring, 30-31.
Gawley, W. G. & Wiggin, S. P. (2016). Water quality monitoring at Acadia National Park: Northeast Temperate Network 2014 summary report. Natural Resource Data Series NPS/NETN/NRDS—2016/1021. National Park Service, Fort Collins, Colorado.
Gibson, A. (2023, March 13). “2022 Visitation Second Highest on Record for Acadia.” Friends of Acadia. https://friendsofacadia.org/stories/2022-visitation-second-highest-on-record-for-acadia/
Gobler, C. J., Davis, T. W., Coyne, K. J., & Boyer, G. L. (2007). Interactive influences of nutrient loading, zooplankton grazing, and microcystin synthetase gene expression on cyanobacterial bloom dynamics in a eutrophic New York lake. Harmful Algae , 6 (1), 119-133.
Gonzalez, P., Wang, F., Notaro, M., Vimont, D. J., & Williams, J. W. (2018). Disproportionate magnitude of climate change in United States national parks. Environmental Research Letters , 13 (10), 104001.
Gsell, A. S., Scharfenberger, U., Özkundakci, D., Walters, A., Hansson, L. A., Janssen, A. B., Nõges, P., Reid, P. C., Schindler, D. E., Van Donk, E., Dakos, V., & Adrian, R. (2016). Evaluating early-warning i ndicators of critical transitions in natural aquatic ecosystems. Proceedings of the National Academy of Sciences , 113 (50), E8089-E8095.
Haei, M., Öquist, M. G., Kreyling, J., Ilstedt, U., & Laudon, H. (2013). Winter climate controls soil carbon dynamics during summer in boreal forests. Environmental Research Letters , 8 (2), 024017.
Hampton, S. E., Galloway, A. W., Powers, S. M., Ozersky, T., Woo, K. H., Batt, R. D., ... & Xenopoulos, M. A. (2017). Ecology under lake ice. Ecology Letters , 20 (1), 98-111.
Hanson, P. C., Carpenter, S. R., Armstrong, D. E., Stanley, E. H., & Kratz, T. K. (2006). Lake dissolved i norganic carbon and dissolved oxygen: changing drivers from days to decades. Ecological Monographs , 76 (3), 343-363.
Harris, G. P. (1980). Temporal and spatial scales in phytoplankton ecology. Mechanisms, methods, models, and management. Canadian Journal of Fisheries and Aquatic Sciences , 37 (5), 877-900.
Harris, T. D., Wilhelm, F. M., Graham, J. L., & Loftin, K. A. (2014). Experimental additions of aluminum sulfate and ammonium nitrate to in situ mesocosms to reduce cyanobacterial biovolume and microcystin concentration. Lake and Reservoir Management , 30 (1), 84-93.
Havens, K. E., James, R. T., East, T. L., & Smith, V. H. (2003). N: P ratios, light limitation, and cyanobacterial dominance in a subtropical lake impacted by non-point source nutrient pollution. Environmental Pollution , 122 (3), 379-390.
Heisler, J., Glibert, P. M., Burkholder, J. M., Anderson, D. M., Cochlan, W., Dennison, W. C., Dortch, Q., Gobler, C. J., Heil, C. A., Humphries, E., Lewitus, A., Magnien, R., Marshall, H. G., Sellner, K., Stockwell, D. A., Stoecker, D. K., & Suddleson, M. (2008). Eutrophication and harmful algal blooms: a scientific consensus. Harmful Algae , 8 (1), 3-13.
Hillebrand, H., Dürselen, C.-D., Kirschtel, D., Pollingher, U., Zohary, T. (1999). Biovolume calculation for pelagic and benthic microalgae. Journal of Phycology , 35(2): 403-424.
Ho, J. C., Michalak, A. M., & Pahlevan, N. (2019). Widespread global increase in intense lake phytoplankton blooms since the 1980s. Nature , 574 (7780), 667-670.
Ho, J. C., & Michalak, A. M. (2017). Phytoplankton blooms in Lake Erie impacted by both long-term and springtime phosphorus loading. Journal of Great Lakes Research , 43 (3), 221-228.
Ho, J. C., & Michalak, A. M. (2020). Exploring temperature and precipitation impacts on harmful algal blooms across continental US lakes. Limnology and Oceanography , 65 (5), 992-1009.
Hodgkins, G. A., James, I. C., & Huntington, T. G. (2002). Historical changes in lake ice ‐ out dates as i ndicators of climate change in New England, 1850–2000. International Journal of Climatology: A Journal of the Royal Meteorological Society , 22 (15), 1819-1827.
Hoef ‐ Emden, K. (2008). Molecular phylogeny of phycocyanin-containing cryptophytes: Evolution of biliproteins and geographical distribution. Journal of Phycology , 44 (4), 985-993.
Hongve, D., Riise, G., & Kristiansen, J. F. (2004). Increased colour and organic acid concentrations in Norwegian forest lakes and drinking water–a result of increased precipitation?. Aquatic Sciences , 66 , 231-238.
Hrycik, A. R., McFarland, S., Morales-Williams, A., & Stockwell, J. D. (2022). Winter severity shapes spring plankton succession in a small, eutrophic lake. Hydrobiologia , 849 (9), 2127-2144.
Hrycik, A. R., & Stockwell, J. D. (2021). Under ‐i ce mesocosms reveal the primacy of light but the i mportance of zooplankton in winter phytoplankton dynamics. Limnology and Oceanography , 66 (2), 481-495.
Huber, V., Wagner, C., Gerten, D., & Adrian, R. (2012). To bloom or not to bloom: contrasting responses of cyanobacteria to recent heat waves explained by critical thresholds of abiotic drivers. Oecologia , 169 , 245-256.
Hutchinson, G. E. (1957). A treatise on limnology, Vol. 1. New York: John Wiley and Sons. 1,015pp.
Intergovernmental Panel on Climate Change (2007). Climate Change 2007: Impacts, Adaptation, and Vulnerability [Parry, M. L., et al. (eds.)]. Cambridge: Cambridge University Press.
Jane, S. F., Hansen, G. J., Kraemer, B. M., Leavitt, P. R., Mincer, J. L., North, R. L., ... & Rose, K. C. (2021). Widespread deoxygenation of temperate lakes. Nature , 594 (7861), 66-70.
Jansen, J., MacIntyre, S., Barrett, D. C., Chin, Y. P., Cortés, A., Forrest, A. L., Hrycik, A. R., Martin, R., McMeans, B. C., Rautio, M., & Schwefel, R. (2021). Winter limnology: How do hydrodynamics and biogeochemistry shape ecosystems under ice?. Journal of Geophysical Research: Biogeosciences , 126 (6), e2020JG006237.
Jenny, J. P., Francus, P., Normandeau, A., Lapointe, F., Perga, M. E., Ojala, A., Schimmelmann, A., & Zolitschka, B. (2016). Global spread of hypoxia in freshwater ecosystems during the last three centuries is caused by rising local human pressure. Global Change Biology , 22 (4), 1481-1489.
Jöhnk , K. D., Huisman, J. E. F., Sharples, J., Sommeijer, B. E. N., Visser, P. M., & Stroom, J. M. (2008). Summer heatwaves promote blooms of harmful cyanobacteria. Global Change Biology , 14 (3), 495-512.
Kanoshina, I., Lips, U., & Leppänen, J. M. (2003). The influence of weather conditions (temperature and wind) on cyanobacterial bloom development in the Gulf of Finland (Baltic Sea). Harmful Algae , 2 (1), 29-41.
Kasinak, J. M. E., Holt, B. M., Chislock, M. F., & Wilson, A. E. (2015). Benchtop fluorometry of phycocyanin as a rapid approach for estimating cyanobacterial biovolume. Journal of Plankton Research , 37 (1), 248-257.
Khan, S. J., Deere, D., Leusch, F. D., Humpage, A., Jenkins, M., & Cunliffe, D. (2015). Extreme weather events: Should drinking water quality management systems adapt to changing risk profiles?. Water Research , 85 , 124-136.
Kidd, E. (2019). Lake Auburn Alum Treatment. Lake Auburn Watershed Protection Commission Newsletter, Summer: 2-3.
Kimura, N., Liu, W. C., Chiu, C. Y., & Kratz, T. K. (2014). Assessing the effects of severe rainstorm-induced mixing on a subtropical, subalpine lake. Environmental Monitoring and Assessment , 186 , 3091-3114.
King, J. R., Shuter, B. J., & Zimmerman, A. P. (1999). Signals of climate trends and extreme events in the thermal stratification pattern of multibasin Lake Opeongo, Ontario. Canadian Journal of Fisheries and Aquatic Sciences , 56 (5), 847-852.
Kirtman, B., Power, S. B., Adedoyin, J. A., Boer, G. J., Bojariu, R., Camilloni, I., Doblas-Reyes, F. J., Fiore, A. M., Kimoto, M.,
Meehl, G. A., Prather, M., Sarr, A., Schär, C., Sutton, R., van Oldenborgh, G. J., Vecchi, G., & Wang, H. J. (2013). Near-term Climate Change: Projections and Predictability. In: Climate Change 2013: The Physical Science Basis. Contribution of Working Group I to the Fifth Assessment Report of the Intergovernmental Panel on Climate Change [Stocker, T.F., Qin, D., Plattner, G., Tignorm, M., Allen,
S. K., Boschung, J., Nauels, A., Xia, Y., Bex, V., & Midgley, P. M. (eds.)]. Cambridge University Press, Cambridge, United Kingdom and New York, NY, USA.
Kopáček, J., Borovec, J., Hejzlar, J., Ulrich, K. U., Norton, S. A., & Amirbahman, A. (2005). Aluminum control of phosphorus sorption by lake sediments. Environmental Science & Technology , 39 (22), 8784-8789.
Kosten, S., Huszar, V. L., Bécares, E., Costa, L. S., van Donk, E., Hansson, L. A., ... & Scheffer, M. (2012). Warmer climates boost cyanobacterial dominance in shallow lakes. Global Change Biology , 18 (1), 118-126.
Kraemer, B. M., Mehner, T., & Adrian, R. (2017). Reconciling the opposing effects of warming on phytoplankton biomass in 188 large lakes. Scientific Reports , 7 (1), 10762.
Kudela, R. M., Berdalet, E., Bernard, S., Burford, M., Fernand, L., Lu, S., Roy, S., Tester, P., Usup, G., Magnien, R., Anderson, D.M., Cembella, A., Chinain, M., Hallegraeff, G., Rguera, B., Zingone, A., Enevoldsen, H., & Urban, E. (2015). Harmful Algal Blooms.
A Scientific Summary for Policy Makers. IOC/UNESCO, Paris (IOC/INF-1320).
Leland, N. (2018). Fundamentals of CyanoCasting: Cost effective monitoring techniques for cyanobacterial surface blooms and cyanotoxin levels. http://lim-tex.com/wp-content/uploads/2018/05/CyanoCasting_Handbook_v18.pdf
Liu, D., Wang, P., Wei, G., Dong, W., & Hui, F. (2013). Removal of algal blooms from freshwater by the coagulation–magnetic separation method. Environmental Science and Pollution Research , 20 , 60-65.
Lofton, M. E., Leach, T. H., Beisner, B. E., & Carey, C. C. (2020). Relative importance of top ‐ down vs. bottom ‐ up control of lake phytoplankton vertical distributions varies among fluorescence ‐ based spectral groups. Limnology and Oceanography , 65 (10), 2485-2501.
Lopez, C. B., Jewett, E. B., Dortch, Q. T. W. B., Walton, B. T., & Hudnell, H. K. (2008). Scientific assessment of freshwater harmful algal blooms. Interagency Working Group on Harmful Algal Blooms, Hypoxia, and Human Health of the Joint Subcommittee on Ocean Science and Technology. Washington, DC.
Lürling, M., Mello, M. M. E., Van Oosterhout, F., de Senerpont Domis, L., & Marinho, M. M. (2018). Response of natural cyanobacteria and algae assemblages to a nutrient pulse and elevated temperature. Frontiers in Microbiology , 9 , 1851.
Lynch, M. (1980). Aphanizomenon blooms: Alternate control and cultivation by Daphnia pulex. In Am. Soc. Limnol. Oceanogr. Spec. Symp (Vol. 3, pp. 299-304).
Magnuson, J. J., Robertson, D. M., Benson, B. J., Wynne, R. H., Livingstone, D. M., Arai, T., Assel, R. A., Barry, R. G., Card, V., Kuusisto, E., Granin, N. G., Prowse, T. D., Stewart, K. M., & Vuglinski, V. S. (2000). Historical trends in lake and river ice cover in the Northern Hemisphere. Science , 289 (5485), 1743-1746.
Magnuson, J. J., Benson, B. J., Jensen, O. P., Clark, T. B., Card, V., Futter, M. N., ... & Stewart, K. M. (2005). Persistence of coherence of ice-off dates for inland lakes across the Laurentian Great Lakes region. Internationale Vereinigung für theoretische und angewandte Limnologie: Verhandlungen , 29 (1), 521-527.
Maine Department of Environmental Protection. (2015). Maine lake assessment quality assurance program plan. Maine Department of Environmental Protection, Augusta, Maine, USA.
Malik, H. I., Warner, K. A., & Saros, J. E. (2018). Comparison of seasonal distribution patterns of Discostella stelligera and Lindavia bodanica in a boreal lake during two years with differing ice-off timing. Diatom Research , 33 (1), 1-11.
McQuaid, N., Zamyadi, A., Prévost, M., Bird, D. F., & Dorner, S. (2011). Use of in vivo phycocyanin fluorescence to monitor potential microcystin-producing cyanobacterial biovolume in a drinking water source. Journal of Environmental Monitoring , 13 (2), 455-463.
Michalak, A. M. (2016). Study role of climate change in extreme threats to water quality. Nature , 535 (7612), 349-350.
Molot, L. A., Dillon, P. J., Clark, B. J., & Neary, B. P. (1992). Predicting end-of-summer oxygen profiles in stratified lakes. Canadian Journal of Fisheries and Aquatic Sciences , 49 (11), 2363-2372.
Molot, L. A., Watson, S. B., Creed, I. F., Trick, C. G., McCabe, S. K., Verschoor, M. J., Sorichetti, R. J., Powe, C., Venkiteswaran, J. J., & Schiff, S. L. (2014). A novel model for cyanobacteria bloom formation: the critical role of anoxia and ferrous iron. Freshwater Biology , 59 (6), 1323-1340.
Monahan, W. B., & Fisichelli, N. A. (2014). Climate exposure of US national parks in a new era of change. PLoS One , 9 (7), e101302.
Murshed, M. F., Aslam, Z., Lewis, R., Chow, C., Wang, D., Drikas, M., & van Leeuwen, J. (2014). Changes in the quality of river water before, during and after a major flood event associated with a La Niña cycle and treatment for drinking purposes. Journal of Environmental Sciences , 26 (10), 1985-1993.
National Park Service. (2018, November 7). Crystal Clear: Algal Toxins in Surface Water at Great Lakes National Parks .
https://www.nps.gov/articles/algal-toxins-in-surface-water-at-great-lakes-national-parks.htm
Nelligan, C., Jeziorski, A., Rühland, K. M., Paterson, A. M., & Smol, J. P. (2019). Long-term trends in hypolimnetic volumes and dissolved oxygen concentrations in Boreal Shield lakes of south-central Ontario, Canada. Canadian Journal of Fisheries and Aquatic Sciences , 76 (12), 2315-2325.
New Hampshire Department of Environmental Services. (2022). Healthy Swimming Mapper.
https://www.des.nh.gov/water/healthy-swimming/healthy-swimming-mapper
North, R. P., North, R. L., Livingstone, D. M., Köster, O., & Kipfer, R. (2014). Long ‐ term changes in hypoxia and soluble reactive phosphorus in the hypolimnion of a large temperate lake: consequences of a climate regime shift. Global Change Biology , 20 (3), 811-823.
Nürnberg, G. K. (1984). The prediction of internal phosphorus load in lakes with anoxic hypolimnia. Limnology and Oceanography , 29 (1), 111-124.
Nürnberg, G. K. (1995). Quantifying anoxia in lakes. Limnology and Oceanography , 40 (6), 1100-1111.
Oliver, S. K., Collins, S. M., Soranno, P. A., Wagner, T., Stanley, E. H., Jones, J. R., Stow, C. A., & Lottig, N. R. (2017). Unexpected stasis in a changing world: Lake nutrient and chlorophyll trends since 1990. Global Change Biology , 23 (12), 5455-5467.
O’Neil, J. M., Davis, T. W., Burford, M. A., & Gobler, C. J. (2012). The rise of harmful cyanobacteria blooms: the potential roles of eutrophication and climate change. Harmful Algae , 14 , 313-334.
O'Reilly, C. M., Sharma, S., Gray, D. K., Hampton, S. E., Read, J. S., Rowley, R. J., ... & Zhang, G.
(2015). Rapid and highly variable warming of lake surface waters around the globe. Geophysical Research Letters , 42 (24), 10-773.
Orihel, D. M., Bird, D. F., Brylinsky, M., Chen, H., Donald, D. B., Huang, D. Y., Giani, A., Kinniburgh, D., Kling, H., Kotak, B. G.,
Leavitt, P. R., Nielsen, C. C., Reedyk, S., Rooney, R. C., Watson, S. B., Zurawell, R. W., & Vinebrooke, R. D. (2012). High microcystin concentrations occur only at low nitrogen-to-phosphorus ratios in nutrient-rich Canadian lakes. Canadian Journal of Fisheries and Aquatic Sciences , 69 (9), 1457-1462.
Orihel, D. M., Baulch, H. M., Casson, N. J., North, R. L., Parsons, C. T., Seckar, D. C., & Venkiteswaran, J. J. (2017). Internal phosphorus loading in Canadian fresh waters: a critical review and data analysis. Canadian Journal of Fisheries and Aquatic Sciences , 74 (12), 2005-2029.
Ortiz, D., Palmer, J., & Wilkinson, G. (2020). Detecting changes in statistical indicators of resilience prior to algal blooms in shallow eutrophic lakes. Ecosphere , 11 (10), e03200.
Otten, T. G., & Paerl, H. W. (2015). Health effects of toxic cyanobacteria in US drinking and recreational waters: our current understanding and proposed direction. Current Environmental Health Reports , 2 , 75-84.
Ozersky, T., Bramburger, A. J., Elgin, A. K., Vanderploeg, H. A., Wang, J., Austin, J. A., ... & Zastepa, A. (2021). The changing face of winter: Lessons and questions from the Laurentian Great Lakes. Journal of Geophysical Research: Biogeosciences , 126, e2021JG006247.
Pace, M. L., Batt, R. D., Buelo, C. D., Carpenter, S. R., Cole, J. J., Kurtzweil, J. T., & Wilkinson, G. M.
(2017). Reversal of a cyanobacterial bloom in response to early warnings. Proceedings of the National Academy of Sciences , 114 (2), 352-357.
Padisak, J. (1992). Seasonal succession of phytoplankton in a large shallow lake (Balaton, Hungary)--A dynamic approach to ecological memory, its possible role and mechanisms. Journal of Ecology , 217-230.
Osgood, R. A. (1988). Lake mixis and internal phosphorus dynamics. Archiv für Hydrobiologie , 629-638.
Paerl, H. W., Tucker, J., & Bland, P. T. (1983). Carotenoid enhancement and its role in maintaining blue ‐ green algal (Microcystis aeruginosa) surface blooms. Limnology and Oceanography , 28 (5), 847-857.
Paerl, H. W. (1988). Nuisance phytoplankton blooms in coastal, estuarine, and inland waters. Limnology and Oceanography , 33 (4part2), 823-843.
Paerl, H. W., Fulton, R. S., Moisander, P. H., & Dyble, J. (2001). Harmful freshwater algal blooms, with an emphasis on cyanobacteria. The Scientific World Journal , 1 , 76-113.
Paerl, H. W. (2017). Controlling cyanobacterial harmful blooms in freshwater ecosystems. Microbial Biotechnology , 10 (5), 1106-1110.
Paerl, H. W., Otten, T. G., & Kudela, R. (2018). Mitigating the expansion of harmful algal blooms across the freshwater-to-marine continuum. Environmental Science and Technology, 52 (10), 5519-5529.
Paerl, H. W., & Huisman, J. (2008). Blooms like it hot. Science , 320 (5872), 57-58.
Paerl, H. W., & Huisman, J. (2009). Climate change: a catalyst for global expansion of harmful cyanobacterial blooms. Environmental Microbiology Reports , 1 (1), 27-37.
Paerl, H. W., & Paul, V. J. (2012). Climate change: Links to global expansion of harmful cyanobacteria. Water Research , 46 (5), 1349-1363.
Peng, G., Martin, R. M., Dearth, S. P., Sun, X., Boyer, G. L., Campagna, S. R., Lin, S., & Wilhelm, S. W. (2018). Seasonally relevant cool temperatures interact with N chemistry to increase microcystins produced in lab cultures of Microcystis aeruginosa NIES-843. Environmental Science & Technology , 52 (7), 4127-4136.
Perello, M. M., Kane, D. D., Golnick, P., Hughes, M. C., Thomas, M. A., & Conroy, J. D. (2017). Effects of l ocal weather variation on water-column stratification and hypoxia in the western, Sandusky, and central basins of Lake Erie. Water , 9 (4), 279.
Persaud, A. D., Paterson, A. M., Ingram, R., Yao, H., & Dillon, P. J. (2014). Potential factors leading to the formation of cyanobacterial scums in a mesotrophic softwater lake in Ontario, Canada. Lake and Reservoir Management , 30 (4), 331-343.
Persaud, A. D., Paterson, A. M., Dillon, P. J., Winter, J. G., Palmer, M., & Somers, K. M. (2015). Forecasting cyanobacteria dominance in Canadian temperate lakes. Journal of Environmental Management , 151 , 343-352.
Peters, D. P., Laney, C. M., Lugo, A. E., Collins, S. L., Driscoll, C. T., Groffman, P. M., Grove, M., Knapp, A., Kratz, T. K., Ohman, M., Waide, R., & Yao, J. (2013). Long-term trends in ecological systems: a basis for understanding responses to global change . US Department of Agriculture, Agricultural Research Service. 378 p. https://www.fs.usda.gov/treesearch/pubs/46691
Pettersson, K., Grust, K., Weyhenmeyer, G., & Blenckner, T. (2003). Seasonality of chlorophyll and nutrients in Lake Erken–effects of weather conditions. Hydrobiologia , 506 , 75-81.
Pilla, R. M., Williamson, C. E., Zhang, J., Smyth, R. L., Lenters, J. D., Brentrup, J. A., ... & Fisher, T. J. (2018). Browning ‐ related decreases in water transparency l ead to long ‐ term increases in surface water temperature and thermal stratification in two small lakes. Journal of Geophysical Research: Biogeosciences , 123 (5), 1651-1665.
Prein, A. F., Rasmussen, R. M., Ikeda, K., Liu, C., Clark, M. P., & Holland, G. J. (2017). The future i ntensification of hourly precipitation extremes. Nature Climate Change , 7 (1), 48-52.
Prepas, E. E., & Vickery, J. (1984). Seasonal changes in total phosphorus and the role of internal loading i n Western Canadian lakes. Internationale Vereinigung für theoretische und angewandte Limnologie: Verhandlungen , 22 (1), 303-308.
R Core Team. (2021). R: A Language and Environment for Statistical Computing. R Foundation for Statistical Computing, Vienna, Austria. http://www.R-project.org/
Read, J. S., Hamilton, D. P., Desai, A. R., Rose, K. C., MacIntyre, S., Lenters, J. D., ... & Wu, C. H. (2012). Lake ‐ size dependency of wind shear and convection as controls on gas exchange. Geophysical Research Letters , 39 (9).
Reichwaldt, E. S., & Ghadouani, A. (2012). Effects of rainfall patterns on toxic cyanobacterial blooms in a changing climate: between simplistic scenarios and complex dynamics. Water Research , 46 (5), 1372-1393.
Reinl, K. L., Brookes, J. D., Carey, C. C., Harris, T. D., Ibelings, B., Morales-Williams, A. M., De Senerpont Domis, L. N., Atkins, K. S., Isles, P. D. F., Mesman, J. P., North, R. L., Rudstam, L. G., Stelzer, J. A. A., Venkiteswaran, J. J., Yokota, K., & Zhan, Q. (2021). Cyanobacterial blooms in oligotrophic lakes: Shifting the high-nutrient paradigm. Freshwater Biology , 66 (9), 1846-1859.
Reinl, K. L., Harris, T. D., North, R. L., Almela, P., Berger, S. A., Bizic, M., ... & Yokota, K. (2023). Blooms also like it cold. Limnology and Oceanography Letters .
Reynolds, C. S., Jaworski, G. H. M., Cmiech, H. A., & Leedale, G. F. (1981). On the annual cycle of the blue-green alga Microcystis aeruginosa Kütz. emend. Elenkin. Philosophical Transactions of the Royal Society of London. B, Biological Sciences , 293 (1068), 419-477.
Reynolds, C.S. (2006). The Ecology of Phytoplankton (Ecology, Biodiversity and Conservation) . Cambridge, UK: Cambridge University Press.
Richardson, D. C., Melles, S. J., Pilla, R. M., Hetherington, A. L., Knoll, L. B., Williamson, C. E., Kraemer, B. J., Jackson, J. R., Long, E. C., Moore, K., Rudstam, L. G., Rusak, J. A., Saros, J. E., Sharma, S., Strock, K. E., Weathers, K. C., & Wigdahl-Perry, C. R. (2017). Transparency, geomorphology and mixing regime explain variability in trends in lake temperature and stratification across Northeastern North America (1975–2014). Water , 9 (6), 442.
Rigosi, A., Carey, C. C., Ibelings, B. W., & Brookes, J. D. (2014). The interaction between climate warming and eutrophication to promote cyanobacteria is dependent on trophic state and varies among taxa. Limnology and Oceanography , 59 (1), 99-114.
Riley, E. T., & Prepas, E. E. (1984). Role of internal phosphorus loading in two shallow, productive lakes i n Alberta, Canada. Canadian Journal of Fisheries and Aquatic Sciences , 41 (6), 845-855.
Robarts, R. D., & Zohary, T. (1987). Temperature effects on photosynthetic capacity, respiration, and growth rates of bloom ‐ forming cyanobacteria. New Zealand Journal of Marine and Freshwater Research , 21 (3), 391-399.
Rohde, E., Pearce, N. J., Young, J., & Xenopoulos, M. A. (2022). Applying early warning indicators to predict critical transitions in a lake undergoing multiple changes. Ecological Applications , 32 (7), e2685.
Romo, S., Soria, J., Fernández, F., Ouahid, Y., & Barón ‐ Solá, Á. (2013). Water residence time and the dynamics of toxic cyanobacteria. Freshwater Biology , 58 (3), 513-522.
Rose, K. C., Winslow, L. A., Read, J. S., & Hansen, G. J. (2016). Climate ‐i nduced warming of lakes can be either amplified or suppressed by trends in water clarity. Limnology and Oceanography Letters , 1 (1), 44-53.
Reuter, W., & Müller, C. (1993). New trends in photobiology: adaptation of the photosynthetic apparatus of cyanobacteria to light and CO2. Journal of Photochemistry and Photobiology B: Biology , 21 (1), 3-27.
Sadro, S., Melack, J. M., Sickman, J. O., & Skeen, K. (2019). Climate warming response of mountain l akes affected by variations in snow. Limnology and Oceanography Letters , 4 (1), 9-17.
Scheffer, M., Carpenter, S., Foley, J. A., Folke, C., & Walker, B. (2001). Catastrophic shifts in ecosystems. Nature , 413 (6856), 591-596.
Scheffer, M., Bascompte, J., Brock, W. A., Brovkin, V., Carpenter, S. R., Dakos, V., Held, H., van Nes, E. H., Rietkerk, M., & Sugihara, G. (2009). Early-warning signals for critical transitions. Nature , 461 (7260), 53-59.
Scheffer, M., Carpenter, S. R., Dakos, V., & van Nes, E. H. (2015). Generic indicators of ecological resilience: inferring the chance of a critical transition. Annual Review of Ecology, Evolution, and Systematics , 46 , 145-167.
Schindler, D. W. (1977). Evolution of phosphorus limitation in lakes: natural mechanisms compensate for deficiencies of nitrogen and carbon in eutrophied lakes. Science , 195 (4275), 260-262.
Schindler, D. W. (2012). The dilemma of controlling cultural eutrophication of lakes. Proceedings of the Royal Society B: Biological Sciences , 279 (1746), 4322-4333.
Schindler, D. W., Carpenter, S. R., Chapra, S. C., Hecky, R. E., & Orihel, D. M. (2016). Reducing phosphorus to curb lake eutrophication is a success. Environmental Science & Technology , 50 (17), 8923-8929.
Schuurman, G. W., Hawkins Hoffman, C., Cole, D. N., Lawrence, D. J., Morton, J. M., Magness, D. R., Cravens, A. E., Covington, S., O’Malley, R., & Fisichelli, N. A. (2020). Resist-accept-direct (RAD)—a framework for the 21st-century natural resource manager. Natural Resource Report
NPS/NRSS/CCRP/NRR—2020/ 2213. National Park Service, Fort Collins, Colorado.
https://doi.org/10.36967/nrr-2283597.
Schuurman, G. (2021). Status of RAD Thinking in NPS. In: Climate Adaptation Using
Resist-Accept-Direct (RAD): Examples in Acadia National Park and Bandelier National Monument . Acadia National Park Science Symposium. https://www.youtube.com/watch?v=fjg4_d-gvj0
Seneviratne, S.I., Zhang, X., Adnan, M., Badi, W., Dereczynski, C., Di Luca, A., Ghosh, S., Iskandar, I., Kossin, J., Lewis, S., Otto, F., Pinto, I., Satoh, M., Vicente-Serrano, S. M., Wehner, M., & Zhou, B. (2021). Weather and Climate Extreme Events in a Changing Climate. In Climate Change 2021: The Physical Science Basis. Contribution of Working Group I to the Sixth Assessment Report of the Intergovernmental Panel on Climate Change [Masson-Delmotte, V., Zhai, P., Pirani, A., Connors, S. L., Péan, C., Berger, S., Caud, N., Chen, Y., Goldfarb, L., Gomis, M. I., Huang, M., Leitzell, K., Lonnoy, E., Matthews, J. B. R., Maycock, T. K., Waterfield, T., Yelekçi, O., Yu, R., Zhou, B. (eds.)]. Cambridge University Press, Cambridge, United Kingdom and New York, NY, USA, pp. 1513–1766, doi:10.1017/9781009157896.013.
Sharma, S., Gray, D. K., Read, J. S., O’Reilly, C. M., Schneider, P., Qudrat, A., ... & Woo, K. H. (2015). A global database of lake surface temperatures collected by in situ and satellite methods from 1985–2009. Scientific Data , 2 (1), 1-19.
Sirota, J., Baiser, B., Gotelli, N. J., & Ellison, A. M. (2013). Organic-matter loading determines regime shifts and alternative states in an aquatic ecosystem. Proceedings of the National Academy of Sciences , 110 (19), 7742-7747.
Smith, V. H., Tilman, G. D., & Nekola, J. C. (1999). Eutrophication: impacts of excess nutrient inputs on freshwater, marine, and terrestrial ecosystems. Environmental Pollution , 100 (1-3), 179-196.
Sorichetti, R. J., Creed, I. F., & Trick, C. G. (2014). The influence of iron, siderophores and refractory DOM on cyanobacterial biomass in oligotrophic lakes. Freshwater Biology , 59 (7), 1423-1436.
Spears, B. M., Futter, M. N., Jeppesen, E., Huser, B. J., Ives, S., Davidson, T. A., ... & Thackeray, S. J. (2017). Ecological resilience in lakes and the conjunction fallacy. Nature Ecology & Evolution , 1 (11), 1616-1624.
Stainsby, E. A., Winter, J. G., Jarjanazi, H., Paterson, A. M., Evans, D. O., & Young, J. D. (2011). Changes in the thermal stability of Lake Simcoe from 1980 to 2008. Journal of Great Lakes Research , 37 , 55-62.
Stoddard, J. L., Van Sickle, J., Herlihy, A. T., Brahney, J., Paulsen, S., Peck, D. V., Mitchell, R., & Pollard, A. I. (2016). Continental-scale increase in lake and stream phosphorus: are oligotrophic systems disappearing in the United States?. Environmental Science & Technology , 50 (7), 3409-3415.
Strock, K. E., Theodore, N., Gawley, W. G., Ellsworth, A. C., & Saros, J. E. (2017). Increasing dissolved organic carbon concentrations in northern boreal lakes: Implications for lake water transparency and thermal structure. Journal of Geophysical Research: Biogeosciences , 122 (5), 1022-1035.
Taranu, Z. E., Zurawell, R. W., Pick, F., & Gregory ‐ Eaves, I. (2012). Predicting cyanobacterial dynamics in the face of global change: the importance of scale and environmental context. Global Change Biology , 18 (12), 3477-3490.
Toffolon, M., Piccolroaz, S., Majone, B., Soja, A. M., Peeters, F., Schmid, M., & Wüest, A. (2014). Prediction of surface temperature in lakes with different morphology using air temperature. Limnology and Oceanography , 59 (6), 2185-2202.
Trimbee, A. M., & Harris, G. P. (1984). Phytoplankton population dynamics of a small reservoir: use of sedimentation traps to quantify the loss of diatoms and recruitment of summer bloom-forming blue-green algae. Journal of Plankton Research , 6 (5), 897-918.
Trimbee, A. M., & Prepas, E. E. (1988). The effect of oxygen depletion on the timing and magnitude of blue-green algal blooms: With 2 figures and 2 tables in the text. Internationale Vereinigung für theoretische und angewandte Limnologie: Verhandlungen , 23 (1), 220-226.
U.S. Environmental Protection Agency. (2000a). Nutrient Criteria Technical Guidance Manual, Lakes and Reservoirs. (1st Ed.). EPA-822-B00-001, April 2000.
U.S. Environmental Protection Agency. (2023, January 20). The Effects: Dead Zones and Harmful Algal Blooms. https://www.epa.gov/nutrientpollution/effects-dead-zones-and-harmful-algal-blooms
U.S. Environmental Protection Agency. (2023, September 28). Cyanobacterial Harmful Algal Blooms
(CyanoHABs) in Water Bodies. https://www.epa.gov/cyanohabs
Üveges, V., Tapolczai, K., Krienitz, L., & Padisák, J. (2012). Photosynthetic characteristics and physiological plasticity of an Aphanizomenon flos-aquae (Cyanobacteria, Nostocaceae) winter bloom in a deep oligo-mesotrophic lake (Lake Stechlin, Germany). Phytoplankton Responses to Human Impacts at Different Scales , 263-272.
Van Nes, E. H., & Scheffer, M. (2007). Slow recovery from perturbations as a generic indicator of a nearby catastrophic shift. The American Naturalist , 169 (6), 738-747.
Vermont Department of Health and Department of Environmental Conservation. (2023). Cyanobacteria (Blue-Green Algae) Tracker .
https://www.healthvermont.gov/environment/tracking/cyanobacteria-blue-green-algae-tracker
Visser, P. M., Ibelings, B. W., Bormans, M., & Huisman, J. (2016). Artificial mixing to control cyanobacterial blooms: a review. Aquatic Ecology , 50 , 423-441.
Vose, R.S., Easterling, D. R., Kunkel, K. E., LeGrande, A. N., & Wehner, M. F. (2017). Temperature changes in the United States. In: Climate Science Special Report: Fourth National Climate Assessment, Volume I [Wuebbles, D.J., Fahey, D. W., Hibbard, K. A., Dokken, D. J., Stewart, B. C., & Maycock, T. K. (eds.)]. U.S. Global Change Research Program, Washington, DC, USA, pp. 185-206, doi:
10.7930/J0N29V45.
Wagner, C., & Adrian, R. (2009). Cyanobacteria dominance: quantifying the effects of climate change. Limnology and Oceanography , 54 (6part2), 2460-2468.
Walsby, A. E. (2001). Determining the photosynthetic productivity of a stratified phytoplankton population. Aquatic Sciences , 63 , 18-43.
Warner, K. A., Fowler, R. A., Northington, R. M., Malik, H. I., McCue, J., & Saros, J. E. (2018). How does changing ice-out affect arctic versus boreal lakes? A comparison using two years with ice-out that differed by more than three weeks. Water , 10 (1), 78.
Warner, K. A., Fowler, R. A., & Saros, J. E. (2020). Differences in the effects of storms on dissolved organic carbon (DOC) in boreal lakes during an early summer storm and an autumn storm. Water , 12 (5), 1452
Warner, K. A., & Saros, J. E. (2019). Variable responses of dissolved organic carbon to precipitation events in boreal drinking water lakes. Water Research , 156 , 315-326.
Watson, S. B., Whitton, B. A., Higgins, S. N., Paerl, H. W., Brooks, B. W., & Wehr, J. D. (2015). Harmful Algal Blooms. In Freshwater Algae of North America (pp. 873-920). Academic Press.
Webster, K. E., Soranno, P. A., Cheruvelil, K. S., Bremigan, M. T., Downing, J. A., Vaux, P. D., ... & Connor, J. (2008). An empirical evaluation of the nutrient ‐ color paradigm for lakes. Limnology and Oceanography , 53 (3), 1137-1148.
Wehr, J. D., Sheath, R. G., & Kociolek, J. P. (Eds.). (2015). Freshwater Algae of North America: Ecology and Classification . Elsevier.
Wetzel, R. G. (2001), Limnology. San Diego: Academic Press, p. 151.
https://doi.org/10.1016/B978-0-08-057439-4.50001-0 ).
Weyhenmeyer, G. A. (2001). Warmer winters: are planktonic algal populations in Sweden's largest lakes affected?. AMBIO: A Journal of the Human Environment , 30 (8), 565-571.
Wiedner, C., Rücker, J., Brüggemann, R., & Nixdorf, B. (2007). Climate change affects timing and size of populations of an invasive cyanobacterium in temperate regions. Oecologia , 152 , 473-484.
Wight, P. (2019, September 19). Maine Lakes Expected To See More Intense, Frequent Toxic Algal Blooms Due To Climate Change . Maine Public.
https://www.mainepublic.org/environment-and-outdoors/2019-09-18/maine-lakes-expected-to-see-more-in tense-frequent-toxic-algal-blooms-due-to-climate-change
Wilkinson, G. M., Carpenter, S. R., Cole, J. J., Pace, M. L., Batt, R. D., Buelo, C. D., & Kurtzweil, J. T.
(2018). Early warning signals precede cyanobacterial blooms in multiple whole ‐l ake experiments. Ecological Monographs , 88 (2), 188-203.
Wilkinson, G. M., Walter, J. A., Buelo, C. D., & Pace, M. L. (2022). No evidence of widespread algal bloom intensification in hundreds of lakes. Frontiers i n Ecology and the Environment , 20 (1), 16-21.
Williamson, C. E., Brentrup, J. A., Zhang, J., Renwick, W. H., Hargreaves, B. R., Knoll, L. B., ... & Rose, K. C. (2014). Lakes as sensors in the landscape: Optical metrics as scalable sentinel responses to climate change. Limnology and Oceanography , 59 (3), 840-850.
Williamson, C. E., Overholt, E. P., Pilla, R. M., Leach, T. H., Brentrup, J. A., Knoll, L. B., Mette, E. M., & Moeller, R. E. (2015). Ecological consequences of long-term browning in lakes. Scientific Reports , 5 (1), 18666
Winslow, L., Read, J., Woolway, R., Brentrup, J., Leach, T., Zwart, J., Albers, S., & Collinge, D. (2019). rLakeAnalyzer: Lake physics tools. R package version 1.11.4.1.
https://CRAN.R-project.org/package=rLakeAnalyzer
Winter, J. G., DeSellas, A. M., Fletcher, R., Heintsch, L., Morley, A., Nakamoto, L., & Utsumi, K. (2011). Algal blooms in Ontario, Canada: increases in reports since 1994. Lake and Reservoir Management , 27 (2), 107-114.
Wong, S., & Hobbs, W. O. (2020). Exploring the Use of Fluorometric Sensors to Monitor Harmful Algal Blooms in Lakes . Publication 20-03-010. Washington State Department of Ecology, Olympia. https://apps. ecology.wa.gov/publications/SummaryPages/2003010.html.
Woolway, R. I., Kraemer, B. M., Lenters, J. D., Merchant, C. J., O’Reilly, C. M., & Sharma, S. (2020). Global lake responses to climate change. Nature Reviews Earth & Environment , 1 (8), 388-403.
Wu, X., Joyce, E. M., & Mason, T. J. (2011). The effects of ultrasound on cyanobacteria. Harmful Algae , 10 (6), 738-743.
Wurtsbaugh, W. A., Paerl, H. W., & Dodds, W. K. (2019). Nutrients, eutrophication and harmful algal blooms along the freshwater to marine continuum. Wiley Interdisciplinary Reviews: Water , 6 (5), e1373.
Wynne, T. T., Stumpf, R. P., Tomlinson, M. C., Schwab, D. J., Watabayashi, G. Y., & Christensen, J. D. (2011). Estimating cyanobacterial bloom transport by coupling remotely sensed imagery and a hydrodynamic model. Ecological Applications , 21 (7), 2709-2721.
Zhang, Y., Loiselle, S., Shi, K., Han, T., Zhang, M., Hu, M., Jing, Y., Lai, L., & Zhan, P. (2021). Wind effects for floating algae dynamics in eutrophic lakes. Remote Sensing , 13 (4), 800.
Zhang, Y., & Prepas, E. E. (1996). Regulation of the dominance of planktonic diatoms and cyanobacteria i n four eutrophic hardwater lakes by nutrients, water column stability, and temperature. Canadian Journal of Fisheries and Aquatic Sciences , 53 (3), 621-633.
Zhao, C. S., Shao, N. F., Yang, S. T., Ren, H., Ge, Y. R., Feng, P., Dong, B. E., & Zhao, Y. (2019). Predicting cyanobacteria bloom occurrence in lakes and reservoirs before blooms occur. Science of the Total Environment , 670 , 837-848.
Zhong, Y., Notaro, M., Vavrus, S. J., & Foster, M. J. (2016). Recent accelerated warming of the Laurentian Great Lakes: Physical drivers. Limnology and Oceanography , 61 (5), 1762-1786.
Agbeti, M. D., & Smol, J. P. (1995). Winter limnology: a comparison of physical, chemical and biological characteristics in two temperate lakes during ice cover. Hydrobiologia, 304 , 221-234.
Alin, S. R., & Johnson, T. C. (2007). Carbon cycling in large lakes of the world: A synthesis of production
American Public Health Association. (2000). Standard Methods for the Examination of Water and Wastewater (20 th Ed.). [Eaton, A., et al. (eds.)]. Washington, D. C., American Public Health Association Press
Amirbahman, A., Norton, S., Fitzgibbon, K., Pavri, F., Bacon, L., & Williams, S. (2016). Maine Lakes: Living on the Edge? [PowerPoint slides]. University of Maine Mitchell Center for Sustainability Solutions Seminar.
Anneville, O., Domaizon, I., Kerimoglu, O., Rimet, F., & Jacquet, S. (2015). Blue-green algae in a “Greenhouse Century”? New insights from field data on climate change impacts on cyanobacteria abundance. Ecosystems, 18 (3), 441-458.
Arheimer, B., Andréasson, J., Fogelberg, S., Johnsson, H., Pers, C. B., & Persson, K. (2005). Climate change impact on water quality: Model results from southern Sweden. AMBIO: A Journal of the Human Environment, 34 (7), 559-566.
Aubriot, L., & Bonilla, S. (2012). Rapid regulation of phosphate uptake in freshwater cyanobacterial blooms. Aquatic Microbial Ecology, 67 (3), 251-263.
Austin, J. A., & Colman, S. M. (2007). Lake Superior summer water temperatures are increasing more rapidly than regional air temperatures: A positive ice ‐ albedo feedback. Geophysical Research Letters, 34 (6).
Bacon, L. (2016). Harmful Algal Blooms and Cyanotoxins in Maine [PowerPoint slides]. Volunteer Lake Monitoring Program Annual Meeting.
Bartow, E. (2023, July 20). Investigating Harmful Algae Blooms with Robin Sleith. Maine EPSCoR. https://umaine.edu/epscor/2023/07/20/investigating-harmful-algae-blooms-with-robin-sleith/
Batt, R. D., Carpenter, S. R., Cole, J. J., Pace, M. L., & Johnson, R. A. (2013). Changes in ecosystem resilience detected in automated measures of ecosystem metabolism during a whole-lake manipulation. Proceedings of the National Academy of Sciences , 110 (43), 17398-17403.
Beall, B. F. N., Twiss, M. R., Smith, D. E., Oyserman, B. O., Rozmarynowycz, M. J., Binding, C. E., Bourbonniere, R. A., Bullerjahn, G. S., Palmer, M. E., Reavie, E. D., Waters, M. K., Woityra, W. C., & McKay, R. M. L. (2016). Ice cover extent drives phytoplankton and bacterial community structure in a l arge north ‐ temperate lake: implications for a warming climate. Environmental Microbiology , 18 (6), 1704-1719.
Beaulieu, M., Pick, F., Palmer, M., Watson, S., Winter, J., Zurawell, R., & Gregory-Eaves, I. (2014). Comparing predictive cyanobacterial models from temperate regions. Canadian Journal of Fisheries and Aquatic Sciences , 71 (12), 1830-1839.
Bestelmeyer, B. T., Ellison, A. M., Fraser, W. R., Gorman, K. B., Holbrook, S. J., Laney, C. M., Ohman, M. D., Peters, D. P. C., Pillsbury, F. C., Rassweiler, A., Schmitt, R. J., & Sharma, S. (2011). Analysis of abrupt transitions in ecological systems. Ecosphere, 2 (12), 1-26.
Beyene, M. T., & Jain, S. (2015). Wintertime weather ‐ climate variability and its links to early spring ice ‐ out i n Maine lakes. Limnology and Oceanography , 60 (6), 1890-1905.
Boeff, K. A., Strock, K. E., & Saros, J. E. (2016). Evaluating planktonic diatom response to climate change across three lakes with differing morphometry. Journal of Paleolimnology , 56 , 33-47.
Blumberg, A. F., & Di Toro, D. M. (1990). Effects of climate warming on dissolved oxygen concentrations i n Lake Erie. Transactions of the American Fisheries Society , 119 (2), 210-223.
Brookes, J. D., Carey, C. C., Hamilton, D. P., Ho, L., van der Linden, L., Renner, R., & Rigosi, A. (2014). Emerging challenges for the drinking water industry. Environmental Science and Technology, 48 (4), 2099-2101.
Brookes, J. D., & Carey, C. C. (2011). Resilience to blooms. Science , 334 (6052), 46-47.
Brothers, S., Köhler, J., Attermeyer, K., Grossart, H. P., Mehner, T., Meyer, N., Scharnweber, K., & Hilt, S. (2014). A feedback loop links brownification and anoxia in a temperate, shallow lake. Limnology and Oceanography , 59 (4), 1388-1398.
Brunberg, A. K., & Blomqvist, P. (2002). Benthic overwintering of Microcystis colonies under different environmental conditions. Journal of Plankton Research , 24 (11), 1247-1252.
Buelo, C. D., Carpenter, S. R., & Pace, M. L. (2018). A modeling analysis of spatial statistical indicators of thresholds for algal blooms. Limnology and Oceanography Letters , 3 (5), 384-392.
Buelo, C. D., Pace, M. L., Carpenter, S. R., Stanley, E. H., Ortiz, D. A., & Ha, D. T. (2022). Evaluating the performance of temporal and spatial early warning statistics of algal blooms. Ecological Applications , 32 (5), e2616.
Burthe, S. J., Henrys, P. A., Mackay, E. B., Spears, B. M., Campbell, R., Carvalho, L., Dudley, B., Gunn, I. D. M., Johns, D. G., Maberly, S. C., May, L., Newell, M. A., Wanless, S., Winfield, I. J., Thackeray, S. J., & Daunt, F. (2016). Do early warning indicators consistently predict nonlinear change in long ‐ term ecological data?. Journal of Applied Ecology , 53 (3), 666-676.
Butitta, V. L., Carpenter, S. R., Loken, L. C., Pace, M. L., & Stanley, E. H. (2017). Spatial early warning signals in a lake manipulation. Ecosphere , 8 (10), e01941.
Cao, H. S., Kong, F. X., Luo, L. C., Shi, X. L., Yang, Z., Zhang, X. F., & Tao, Y. (2006). Effects of wind and wind-induced waves on vertical phytoplankton distribution and surface blooms of Microcystis aeruginosa i n Lake Taihu. Journal of Freshwater Ecology , 21 (2), 231-238.
Carlson, R. E., & Simpson, J. (1996). A biomass based trophic state index. North American Lake Management Society .
Carey, C. C., Ewing, H. A., Cottingham, K. L., Weathers, K. C., Thomas, R. Q., & Haney, J. F. (2012b). Occurrence and toxicity of the cyanobacterium Gloeotrichia echinulata in low-nutrient lakes in the northeastern United States. Aquatic Ecology , 46 , 395-409.
Carey, C. C., Ibelings, B. W., Hoffmann, E. P., Hamilton, D. P., & Brookes, J. D. (2012a). Eco-physiological adaptations that favour freshwater cyanobacteria in a changing climate. Water Research , 46 (5), 1394-1407.
Carey, C. C., Woelmer, W. M., Lofton, M. E., Figueiredo, R. J., Bookout, B. J., Corrigan, R. S., Daneshmand, V., Hounshell, A. G., Howard, D. W., Lewis, A. S. L., McClure, R. P., Wander, H. L., Ward, N. K., & Thomas, R. Q. (2022). Advancing lake and reservoir water quality management with near-term, i terative ecological forecasting. Inland Waters , 12 (1), 107-120.
Carpenter, S. R., Ludwig, D., & Brock, W. A. (1999). Management of eutrophication for lakes subject to potentially irreversible change. Ecological Applications , 9 (3), 751-771.
Wilkinson, G. M., Carpenter, S. R., Cole, J. J., Pace, M. L., Batt, R. D., Buelo, C. D., & Kurtzweil, J. T. (2018). Early warning signals precede cyanobacterial blooms in multiple whole ‐l ake experiments. Ecological Monographs , 88 (2), 188-203.
Cavaliere, E., Fournier, I. B., Hazuková, V., Rue, G. P., Sadro, S., Berger, S. A., Cotner, J. B., Dugan, H. A., Hampton, S. E., Lottig, N. R., McMeans, B. C., Ozersky, T., Powers, S. M., Rautio, M., & O'Reilly, C.
M. (2021). The lake ice continuum concept: influence of winter conditions on energy and ecosystem dynamics. Journal of Geophysical Research: Biogeosciences , 126 (11), e2020JG006165.
Center for Disease Control and Prevention. (2023). Harmful Algal Bloom (HAB)-Associated Illness. https://www.cdc.gov/habs/index.html
Chapra, S. C., Boehlert, B., Fant, C., Bierman Jr, V. J., Henderson, J., Mills, D., ... & Paerl, H. W. (2017). Climate change impacts on harmful algal blooms in US freshwaters: a screening-level assessment. Environmental Science & Technology , 51 (16), 8933-8943.
Chorus, I., & Welker, M. (Eds.). (2021). Toxic Cyanobacteria in Water: A Guide to Their Public Health Consequences, Monitoring and Management (2nd ed.). CRC Press. https://doi.org/10.1201/9781003081449
Clilverd, H., White, D., & Lilly, M. (2009). Chemical and Physical Controls on the Oxygen Regime of Ice ‐ Covered Arctic Lakes and Reservoirs 1. JAWRA Journal of the American Water Resources Association , 45 (2), 500-511.
Coles, J. F., & Jones, R. C. (2000). Effect of temperature on photosynthesis ‐l ight response and growth of four phytoplankton species isolated from a tidal freshwater river. Journal of Phycology , 36 (1), 7-16.
Cottingham, K. L., Ewing, H. A., Greer, M. L., Carey, C. C., & Weathers, K. C. (2015). Cyanobacteria as biological drivers of lake nitrogen and phosphorus cycling. Ecosphere , 6 (1), 1-19.
Couture, S., Houle, D., & Gagnon, C. (2012). Increases of dissolved organic carbon in temperate and boreal lakes in Quebec, Canada. Environmental Science and Pollution Research , 19 (2), 361-371.
Couture, R. M., de Wit, H. A., Tominaga, K., Kiuru, P., & Markelov, I. (2015). Oxygen dynamics in a boreal l ake responds to long ‐ term changes in climate, ice phenology, and DOC inputs. Journal of Geophysical Research: Biogeosciences , 120 (11), 2441-2456.
Creed, I. F., Bergström, A. K., Trick, C. G., Grimm, N. B., Hessen, D. O., Karlsson, J., Kidd, K. A., Kritzberg, E., McKnight, D. M., Freeman, E. C., Senar, O. E., Andersson, A., Ask, J., Berggren, M., Cherif, M., Giesler, R., Hotchkiss, E. R., Kortelainen, P., Palta, M. M., Vrede, T., & Weyhenmeyer, G. A. (2018). Global change ‐ driven effects on dissolved organic matter composition: Implications for food webs of northern lakes. Global Change Biology , 24 (8), 3692-3714.
Daggett, C. T., Saros, J. E., Lafrancois, B. M., Simon, K. S., & Amirbahman, A. (2015). Effects of i ncreased concentrations of inorganic nitrogen and dissolved organic matter on phytoplankton in boreal l akes with differing nutrient limitation patterns. Aquatic Sciences , 77 , 511-521.
Dakos, V., Carpenter, S. R., van Nes, E. H., & Scheffer, M. (2015). Resilience indicators: prospects and l imitations for early warnings of regime shifts. Philosophical Transactions of the Royal Society B: Biological Sciences , 370 (1659), 20130263.
Dawson, T. P., Jackson, S. T., House, J. I., Prentice, I. C., & Mace, G. M. (2011). Beyond predictions: biodiversity conservation in a changing climate. Science , 332 (6025), 53-58.
Deeds, J., Amirbahman, A., Norton, S. A., Suitor, D. G., & Bacon, L. C. (2021). Predicting anoxia in l ow ‐ nutrient temperate lakes. Ecological Applications , 31 (6), e02361.
Deng, J., Qin, B., Paerl, H. W., Zhang, Y., Ma, J., & Chen, Y. (2014). Earlier and warmer springs increase cyanobacterial (Microcystis spp.) blooms in subtropical Lake Taihu, China. Freshwater Biology , 59 (5), 1076-1085.
Downing, J. A., Watson, S. B., & McCauley, E. (2001). Predicting cyanobacteria dominance in lakes. Canadian Journal of Fisheries and Aquatic Sciences , 58 (10), 1905-1908.
Dugan, N. R., Smith, S. J., & Sanan, T. T. (2018). Impacts of potassium permanganate and powdered activated carbon on cyanotoxin release. Journal ‐ American Water Works Association , 110 (11), E31-E42.
Easterling, D. R., Kunkel, K. E., Arnold, J. R., Knutson, T., LeGrande, A. N., Leung, L. R., Vose, R. S., Waliser, D. E., & Wehner, M. F. (2017). Precipitation change in the United States. In: Climate Science Special Report: Fourth National Climate Assessment, Volume I [Wuebbles, D.J., Fahey, D. W., Hibbard, K. A., Dokken, D. J., Stewart, B. C., & Maycock, T. K. (eds.)]. U.S. Global Change Research Program, Washington, DC, USA, pp. 207-230, doi: 10.7930/J0H993CC.
Elliott, J. A., Jones, I. D., & Thackeray, S. J. (2006). Testing the sensitivity of phytoplankton communities to changes in water temperature and nutrient load, in a temperate lake. Hydrobiologia , 559 , 401-411.
Elliott, J. A. (2012). Is the future blue-green? A review of the current model predictions of how climate change could affect pelagic freshwater cyanobacteria. Water Research , 46 (5), 1364-1371.
Ellis, A. W., & Greene, T. R. (2019). Synoptic climate evidence of a late-twentieth century change to earlier spring ice-out on Maine Lakes, USA. Climatic Change , 153 (3), 323-339.
Elser, J. J. (1999). The pathway to noxious cyanobacteria blooms in lakes: the food web as the final turn. Freshwater Biology , 42 (3), 537-543.
Fee, E. J., Hecky, R. E., Kasian, S. E. M., & Cruikshank, D. R. (1996). Effects of lake size, water clarity, and climatic variability on mixing depths in Canadian Shield lakes. Limnology and Oceanography , 41 (5), 912-920.
Fernandez, I.J., Schmitt, C., Birkel, S., Stancioff, E., Pershing, A., Kelley, J., Runge, J., Jacobson, G., & Mayewski, P. (2015). Maine’s Climate Future: 2015 Update. Orono, ME: University of Maine. 24pp
Fernandez, I., Birkel, S., Schmitt, C., Simonson, J., Lyon, B., Pershing, A., Stancioff, E., Jacobson, G., & and Mayewski, P. (2020). Maine’s Climate Future 2020 Update. Orono, ME: University of Maine. climatechange.umaine.edu/climate-matters/maines-climate-future/
Finstad, A. G., Helland, I. P., Ugedal, O., Hesthagen, T., & Hessen, D. O. (2014). Unimodal response of fish yield to dissolved organic carbon. Ecology Letters , 17 (1), 36-43.
Fisichelli, N.A., Peters, M., Iverson, L., Matthews, S., & Hawkins Hoffman, C. (2013). Climate change and forests of the Acadia National Park region: Projected changes in habitat suitability for 83 tree species. Natural Resource Report NPS/ACAD/NRR—2013/733. National Park Service, Fort Collins, Colorado.
Fisichelli N. A., Schuurman, G. W., Symstad, A. J., Ray, A., Miller, B., Cross, M., & Rowland, E. (2016b). Resource Management and Operations in Southwest South Dakota: Climate Change Scenario Planning Workshop Summary January 20–21 2016, Rapid City, SD. National Park Service. Report no.
NPS/NRSS/NRR—2016/1289.
Freeman, E. C., Creed, I. F., Jones, B., & Bergström, A. K. (2020). Global changes may be promoting a rise in select cyanobacteria in nutrient ‐ poor northern l akes. Global Change Biology , 26 (9), 4966-4987.
Foley, B., Jones, I. D., Maberly, S. C., & Rippey, B. (2012). Long ‐ term changes in oxygen depletion i n a small temperate lake: effects of climate change and eutrophication. Freshwater Biology , 57 (2), 278-289.
Fowler, R. A., Warner, K. A., Gawley, W. G., & Saros, J. E. (2022). Paleolimnological comparison of algal changes in a clear-versus a brown-water lake over the last two centuries in the northeastern USA. Journal of Paleolimnology , 67 (3), 289-305.
Gawley, B. (2023). A Little Ice Would Be Nice. ACADIA Journal, Winter/Spring, 30-31.
Gawley, W. G. & Wiggin, S. P. (2016). Water quality monitoring at Acadia National Park: Northeast Temperate Network 2014 summary report. Natural Resource Data Series NPS/NETN/NRDS—2016/1021. National Park Service, Fort Collins, Colorado.
Gibson, A. (2023, March 13). “2022 Visitation Second Highest on Record for Acadia.” Friends of Acadia. https://friendsofacadia.org/stories/2022-visitation-second-highest-on-record-for-acadia/
Gobler, C. J., Davis, T. W., Coyne, K. J., & Boyer, G. L. (2007). Interactive influences of nutrient loading, zooplankton grazing, and microcystin synthetase gene expression on cyanobacterial bloom dynamics in a eutrophic New York lake. Harmful Algae , 6 (1), 119-133.
Gonzalez, P., Wang, F., Notaro, M., Vimont, D. J., & Williams, J. W. (2018). Disproportionate magnitude of climate change in United States national parks. Environmental Research Letters , 13 (10), 104001.
Gsell, A. S., Scharfenberger, U., Özkundakci, D., Walters, A., Hansson, L. A., Janssen, A. B., Nõges, P., Reid, P. C., Schindler, D. E., Van Donk, E., Dakos, V., & Adrian, R. (2016). Evaluating early-warning i ndicators of critical transitions in natural aquatic ecosystems. Proceedings of the National Academy of Sciences , 113 (50), E8089-E8095.
Haei, M., Öquist, M. G., Kreyling, J., Ilstedt, U., & Laudon, H. (2013). Winter climate controls soil carbon dynamics during summer in boreal forests. Environmental Research Letters , 8 (2), 024017.
Hampton, S. E., Galloway, A. W., Powers, S. M., Ozersky, T., Woo, K. H., Batt, R. D., ... & Xenopoulos, M. A. (2017). Ecology under lake ice. Ecology Letters , 20 (1), 98-111.
Hanson, P. C., Carpenter, S. R., Armstrong, D. E., Stanley, E. H., & Kratz, T. K. (2006). Lake dissolved i norganic carbon and dissolved oxygen: changing drivers from days to decades. Ecological Monographs , 76 (3), 343-363.
Harris, G. P. (1980). Temporal and spatial scales in phytoplankton ecology. Mechanisms, methods, models, and management. Canadian Journal of Fisheries and Aquatic Sciences , 37 (5), 877-900.
Harris, T. D., Wilhelm, F. M., Graham, J. L., & Loftin, K. A. (2014). Experimental additions of aluminum sulfate and ammonium nitrate to in situ mesocosms to reduce cyanobacterial biovolume and microcystin concentration. Lake and Reservoir Management , 30 (1), 84-93.
Havens, K. E., James, R. T., East, T. L., & Smith, V. H. (2003). N: P ratios, light limitation, and cyanobacterial dominance in a subtropical lake impacted by non-point source nutrient pollution. Environmental Pollution , 122 (3), 379-390.
Heisler, J., Glibert, P. M., Burkholder, J. M., Anderson, D. M., Cochlan, W., Dennison, W. C., Dortch, Q., Gobler, C. J., Heil, C. A., Humphries, E., Lewitus, A., Magnien, R., Marshall, H. G., Sellner, K., Stockwell, D. A., Stoecker, D. K., & Suddleson, M. (2008). Eutrophication and harmful algal blooms: a scientific consensus. Harmful Algae , 8 (1), 3-13.
Hillebrand, H., Dürselen, C.-D., Kirschtel, D., Pollingher, U., Zohary, T. (1999). Biovolume calculation for pelagic and benthic microalgae. Journal of Phycology , 35(2): 403-424.
Ho, J. C., Michalak, A. M., & Pahlevan, N. (2019). Widespread global increase in intense lake phytoplankton blooms since the 1980s. Nature , 574 (7780), 667-670.
Ho, J. C., & Michalak, A. M. (2017). Phytoplankton blooms in Lake Erie impacted by both long-term and springtime phosphorus loading. Journal of Great Lakes Research , 43 (3), 221-228.
Ho, J. C., & Michalak, A. M. (2020). Exploring temperature and precipitation impacts on harmful algal blooms across continental US lakes. Limnology and Oceanography , 65 (5), 992-1009.
Hodgkins, G. A., James, I. C., & Huntington, T. G. (2002). Historical changes in lake ice ‐ out dates as i ndicators of climate change in New England, 1850–2000. International Journal of Climatology: A Journal of the Royal Meteorological Society , 22 (15), 1819-1827.
Hoef ‐ Emden, K. (2008). Molecular phylogeny of phycocyanin-containing cryptophytes: Evolution of biliproteins and geographical distribution. Journal of Phycology , 44 (4), 985-993.
Hongve, D., Riise, G., & Kristiansen, J. F. (2004). Increased colour and organic acid concentrations in Norwegian forest lakes and drinking water–a result of increased precipitation?. Aquatic Sciences , 66 , 231-238.
Hrycik, A. R., McFarland, S., Morales-Williams, A., & Stockwell, J. D. (2022). Winter severity shapes spring plankton succession in a small, eutrophic lake. Hydrobiologia , 849 (9), 2127-2144.
Hrycik, A. R., & Stockwell, J. D. (2021). Under ‐i ce mesocosms reveal the primacy of light but the i mportance of zooplankton in winter phytoplankton dynamics. Limnology and Oceanography , 66 (2), 481-495.
Huber, V., Wagner, C., Gerten, D., & Adrian, R. (2012). To bloom or not to bloom: contrasting responses of cyanobacteria to recent heat waves explained by critical thresholds of abiotic drivers. Oecologia , 169 , 245-256.
Hutchinson, G. E. (1957). A treatise on limnology, Vol. 1. New York: John Wiley and Sons. 1,015pp.
Intergovernmental Panel on Climate Change (2007). Climate Change 2007: Impacts, Adaptation, and Vulnerability [Parry, M. L., et al. (eds.)]. Cambridge: Cambridge University Press.
Jane, S. F., Hansen, G. J., Kraemer, B. M., Leavitt, P. R., Mincer, J. L., North, R. L., ... & Rose, K. C. (2021). Widespread deoxygenation of temperate lakes. Nature , 594 (7861), 66-70.
Jansen, J., MacIntyre, S., Barrett, D. C., Chin, Y. P., Cortés, A., Forrest, A. L., Hrycik, A. R., Martin, R., McMeans, B. C., Rautio, M., & Schwefel, R. (2021). Winter limnology: How do hydrodynamics and biogeochemistry shape ecosystems under ice?. Journal of Geophysical Research: Biogeosciences , 126 (6), e2020JG006237.
Jenny, J. P., Francus, P., Normandeau, A., Lapointe, F., Perga, M. E., Ojala, A., Schimmelmann, A., & Zolitschka, B. (2016). Global spread of hypoxia in freshwater ecosystems during the last three centuries is caused by rising local human pressure. Global Change Biology , 22 (4), 1481-1489.
Jöhnk , K. D., Huisman, J. E. F., Sharples, J., Sommeijer, B. E. N., Visser, P. M., & Stroom, J. M. (2008). Summer heatwaves promote blooms of harmful cyanobacteria. Global Change Biology , 14 (3), 495-512.
Kanoshina, I., Lips, U., & Leppänen, J. M. (2003). The influence of weather conditions (temperature and wind) on cyanobacterial bloom development in the Gulf of Finland (Baltic Sea). Harmful Algae , 2 (1), 29-41.
Kasinak, J. M. E., Holt, B. M., Chislock, M. F., & Wilson, A. E. (2015). Benchtop fluorometry of phycocyanin as a rapid approach for estimating cyanobacterial biovolume. Journal of Plankton Research , 37 (1), 248-257.
Khan, S. J., Deere, D., Leusch, F. D., Humpage, A., Jenkins, M., & Cunliffe, D. (2015). Extreme weather events: Should drinking water quality management systems adapt to changing risk profiles?. Water Research , 85 , 124-136.
Kidd, E. (2019). Lake Auburn Alum Treatment. Lake Auburn Watershed Protection Commission Newsletter, Summer: 2-3.
Kimura, N., Liu, W. C., Chiu, C. Y., & Kratz, T. K. (2014). Assessing the effects of severe rainstorm-induced mixing on a subtropical, subalpine lake. Environmental Monitoring and Assessment , 186 , 3091-3114.
King, J. R., Shuter, B. J., & Zimmerman, A. P. (1999). Signals of climate trends and extreme events in the thermal stratification pattern of multibasin Lake Opeongo, Ontario. Canadian Journal of Fisheries and Aquatic Sciences , 56 (5), 847-852.
Kirtman, B., Power, S. B., Adedoyin, J. A., Boer, G. J., Bojariu, R., Camilloni, I., Doblas-Reyes, F. J., Fiore, A. M., Kimoto, M.,
Meehl, G. A., Prather, M., Sarr, A., Schär, C., Sutton, R., van Oldenborgh, G. J., Vecchi, G., & Wang, H. J. (2013). Near-term Climate Change: Projections and Predictability. In: Climate Change 2013: The Physical Science Basis. Contribution of Working Group I to the Fifth Assessment Report of the Intergovernmental Panel on Climate Change [Stocker, T.F., Qin, D., Plattner, G., Tignorm, M., Allen,
S. K., Boschung, J., Nauels, A., Xia, Y., Bex, V., & Midgley, P. M. (eds.)]. Cambridge University Press, Cambridge, United Kingdom and New York, NY, USA.
Kopáček, J., Borovec, J., Hejzlar, J., Ulrich, K. U., Norton, S. A., & Amirbahman, A. (2005). Aluminum control of phosphorus sorption by lake sediments. Environmental Science & Technology , 39 (22), 8784-8789.
Kosten, S., Huszar, V. L., Bécares, E., Costa, L. S., van Donk, E., Hansson, L. A., ... & Scheffer, M. (2012). Warmer climates boost cyanobacterial dominance in shallow lakes. Global Change Biology , 18 (1), 118-126.
Kraemer, B. M., Mehner, T., & Adrian, R. (2017). Reconciling the opposing effects of warming on phytoplankton biomass in 188 large lakes. Scientific Reports , 7 (1), 10762.
Kudela, R. M., Berdalet, E., Bernard, S., Burford, M., Fernand, L., Lu, S., Roy, S., Tester, P., Usup, G., Magnien, R., Anderson, D.M., Cembella, A., Chinain, M., Hallegraeff, G., Rguera, B., Zingone, A., Enevoldsen, H., & Urban, E. (2015). Harmful Algal Blooms.
A Scientific Summary for Policy Makers. IOC/UNESCO, Paris (IOC/INF-1320).
Leland, N. (2018). Fundamentals of CyanoCasting: Cost effective monitoring techniques for cyanobacterial surface blooms and cyanotoxin levels. http://lim-tex.com/wp-content/uploads/2018/05/CyanoCasting_Handbook_v18.pdf
Liu, D., Wang, P., Wei, G., Dong, W., & Hui, F. (2013). Removal of algal blooms from freshwater by the coagulation–magnetic separation method. Environmental Science and Pollution Research , 20 , 60-65.
Lofton, M. E., Leach, T. H., Beisner, B. E., & Carey, C. C. (2020). Relative importance of top ‐ down vs. bottom ‐ up control of lake phytoplankton vertical distributions varies among fluorescence ‐ based spectral groups. Limnology and Oceanography , 65 (10), 2485-2501.
Lopez, C. B., Jewett, E. B., Dortch, Q. T. W. B., Walton, B. T., & Hudnell, H. K. (2008). Scientific assessment of freshwater harmful algal blooms. Interagency Working Group on Harmful Algal Blooms, Hypoxia, and Human Health of the Joint Subcommittee on Ocean Science and Technology. Washington, DC.
Lürling, M., Mello, M. M. E., Van Oosterhout, F., de Senerpont Domis, L., & Marinho, M. M. (2018). Response of natural cyanobacteria and algae assemblages to a nutrient pulse and elevated temperature. Frontiers in Microbiology , 9 , 1851.
Lynch, M. (1980). Aphanizomenon blooms: Alternate control and cultivation by Daphnia pulex. In Am. Soc. Limnol. Oceanogr. Spec. Symp (Vol. 3, pp. 299-304).
Magnuson, J. J., Robertson, D. M., Benson, B. J., Wynne, R. H., Livingstone, D. M., Arai, T., Assel, R. A., Barry, R. G., Card, V., Kuusisto, E., Granin, N. G., Prowse, T. D., Stewart, K. M., & Vuglinski, V. S. (2000). Historical trends in lake and river ice cover in the Northern Hemisphere. Science , 289 (5485), 1743-1746.
Magnuson, J. J., Benson, B. J., Jensen, O. P., Clark, T. B., Card, V., Futter, M. N., ... & Stewart, K. M. (2005). Persistence of coherence of ice-off dates for inland lakes across the Laurentian Great Lakes region. Internationale Vereinigung für theoretische und angewandte Limnologie: Verhandlungen , 29 (1), 521-527.
Maine Department of Environmental Protection. (2015). Maine lake assessment quality assurance program plan. Maine Department of Environmental Protection, Augusta, Maine, USA.
Malik, H. I., Warner, K. A., & Saros, J. E. (2018). Comparison of seasonal distribution patterns of Discostella stelligera and Lindavia bodanica in a boreal lake during two years with differing ice-off timing. Diatom Research , 33 (1), 1-11.
McQuaid, N., Zamyadi, A., Prévost, M., Bird, D. F., & Dorner, S. (2011). Use of in vivo phycocyanin fluorescence to monitor potential microcystin-producing cyanobacterial biovolume in a drinking water source. Journal of Environmental Monitoring , 13 (2), 455-463.
Michalak, A. M. (2016). Study role of climate change in extreme threats to water quality. Nature , 535 (7612), 349-350.
Molot, L. A., Dillon, P. J., Clark, B. J., & Neary, B. P. (1992). Predicting end-of-summer oxygen profiles in stratified lakes. Canadian Journal of Fisheries and Aquatic Sciences , 49 (11), 2363-2372.
Molot, L. A., Watson, S. B., Creed, I. F., Trick, C. G., McCabe, S. K., Verschoor, M. J., Sorichetti, R. J., Powe, C., Venkiteswaran, J. J., & Schiff, S. L. (2014). A novel model for cyanobacteria bloom formation: the critical role of anoxia and ferrous iron. Freshwater Biology , 59 (6), 1323-1340.
Monahan, W. B., & Fisichelli, N. A. (2014). Climate exposure of US national parks in a new era of change. PLoS One , 9 (7), e101302.
Murshed, M. F., Aslam, Z., Lewis, R., Chow, C., Wang, D., Drikas, M., & van Leeuwen, J. (2014). Changes in the quality of river water before, during and after a major flood event associated with a La Niña cycle and treatment for drinking purposes. Journal of Environmental Sciences , 26 (10), 1985-1993.
National Park Service. (2018, November 7). Crystal Clear: Algal Toxins in Surface Water at Great Lakes National Parks .
https://www.nps.gov/articles/algal-toxins-in-surface-water-at-great-lakes-national-parks.htm
Nelligan, C., Jeziorski, A., Rühland, K. M., Paterson, A. M., & Smol, J. P. (2019). Long-term trends in hypolimnetic volumes and dissolved oxygen concentrations in Boreal Shield lakes of south-central Ontario, Canada. Canadian Journal of Fisheries and Aquatic Sciences , 76 (12), 2315-2325.
New Hampshire Department of Environmental Services. (2022). Healthy Swimming Mapper.
https://www.des.nh.gov/water/healthy-swimming/healthy-swimming-mapper
North, R. P., North, R. L., Livingstone, D. M., Köster, O., & Kipfer, R. (2014). Long ‐ term changes in hypoxia and soluble reactive phosphorus in the hypolimnion of a large temperate lake: consequences of a climate regime shift. Global Change Biology , 20 (3), 811-823.
Nürnberg, G. K. (1984). The prediction of internal phosphorus load in lakes with anoxic hypolimnia. Limnology and Oceanography , 29 (1), 111-124.
Nürnberg, G. K. (1995). Quantifying anoxia in lakes. Limnology and Oceanography , 40 (6), 1100-1111.
Oliver, S. K., Collins, S. M., Soranno, P. A., Wagner, T., Stanley, E. H., Jones, J. R., Stow, C. A., & Lottig, N. R. (2017). Unexpected stasis in a changing world: Lake nutrient and chlorophyll trends since 1990. Global Change Biology , 23 (12), 5455-5467.
O’Neil, J. M., Davis, T. W., Burford, M. A., & Gobler, C. J. (2012). The rise of harmful cyanobacteria blooms: the potential roles of eutrophication and climate change. Harmful Algae , 14 , 313-334.
O'Reilly, C. M., Sharma, S., Gray, D. K., Hampton, S. E., Read, J. S., Rowley, R. J., ... & Zhang, G.
(2015). Rapid and highly variable warming of lake surface waters around the globe. Geophysical Research Letters , 42 (24), 10-773.
Orihel, D. M., Bird, D. F., Brylinsky, M., Chen, H., Donald, D. B., Huang, D. Y., Giani, A., Kinniburgh, D., Kling, H., Kotak, B. G.,
Leavitt, P. R., Nielsen, C. C., Reedyk, S., Rooney, R. C., Watson, S. B., Zurawell, R. W., & Vinebrooke, R. D. (2012). High microcystin concentrations occur only at low nitrogen-to-phosphorus ratios in nutrient-rich Canadian lakes. Canadian Journal of Fisheries and Aquatic Sciences , 69 (9), 1457-1462.
Orihel, D. M., Baulch, H. M., Casson, N. J., North, R. L., Parsons, C. T., Seckar, D. C., & Venkiteswaran, J. J. (2017). Internal phosphorus loading in Canadian fresh waters: a critical review and data analysis. Canadian Journal of Fisheries and Aquatic Sciences , 74 (12), 2005-2029.
Ortiz, D., Palmer, J., & Wilkinson, G. (2020). Detecting changes in statistical indicators of resilience prior to algal blooms in shallow eutrophic lakes. Ecosphere , 11 (10), e03200.
Otten, T. G., & Paerl, H. W. (2015). Health effects of toxic cyanobacteria in US drinking and recreational waters: our current understanding and proposed direction. Current Environmental Health Reports , 2 , 75-84.
Ozersky, T., Bramburger, A. J., Elgin, A. K., Vanderploeg, H. A., Wang, J., Austin, J. A., ... & Zastepa, A. (2021). The changing face of winter: Lessons and questions from the Laurentian Great Lakes. Journal of Geophysical Research: Biogeosciences , 126, e2021JG006247.
Pace, M. L., Batt, R. D., Buelo, C. D., Carpenter, S. R., Cole, J. J., Kurtzweil, J. T., & Wilkinson, G. M.
(2017). Reversal of a cyanobacterial bloom in response to early warnings. Proceedings of the National Academy of Sciences , 114 (2), 352-357.
Padisak, J. (1992). Seasonal succession of phytoplankton in a large shallow lake (Balaton, Hungary)--A dynamic approach to ecological memory, its possible role and mechanisms. Journal of Ecology , 217-230.
Osgood, R. A. (1988). Lake mixis and internal phosphorus dynamics. Archiv für Hydrobiologie , 629-638.
Paerl, H. W., Tucker, J., & Bland, P. T. (1983). Carotenoid enhancement and its role in maintaining blue ‐ green algal (Microcystis aeruginosa) surface blooms. Limnology and Oceanography , 28 (5), 847-857.
Paerl, H. W. (1988). Nuisance phytoplankton blooms in coastal, estuarine, and inland waters. Limnology and Oceanography , 33 (4part2), 823-843.
Paerl, H. W., Fulton, R. S., Moisander, P. H., & Dyble, J. (2001). Harmful freshwater algal blooms, with an emphasis on cyanobacteria. The Scientific World Journal , 1 , 76-113.
Paerl, H. W. (2017). Controlling cyanobacterial harmful blooms in freshwater ecosystems. Microbial Biotechnology , 10 (5), 1106-1110.
Paerl, H. W., Otten, T. G., & Kudela, R. (2018). Mitigating the expansion of harmful algal blooms across the freshwater-to-marine continuum. Environmental Science and Technology, 52 (10), 5519-5529.
Paerl, H. W., & Huisman, J. (2008). Blooms like it hot. Science , 320 (5872), 57-58.
Paerl, H. W., & Huisman, J. (2009). Climate change: a catalyst for global expansion of harmful cyanobacterial blooms. Environmental Microbiology Reports , 1 (1), 27-37.
Paerl, H. W., & Paul, V. J. (2012). Climate change: Links to global expansion of harmful cyanobacteria. Water Research , 46 (5), 1349-1363.
Peng, G., Martin, R. M., Dearth, S. P., Sun, X., Boyer, G. L., Campagna, S. R., Lin, S., & Wilhelm, S. W. (2018). Seasonally relevant cool temperatures interact with N chemistry to increase microcystins produced in lab cultures of Microcystis aeruginosa NIES-843. Environmental Science & Technology , 52 (7), 4127-4136.
Perello, M. M., Kane, D. D., Golnick, P., Hughes, M. C., Thomas, M. A., & Conroy, J. D. (2017). Effects of l ocal weather variation on water-column stratification and hypoxia in the western, Sandusky, and central basins of Lake Erie. Water , 9 (4), 279.
Persaud, A. D., Paterson, A. M., Ingram, R., Yao, H., & Dillon, P. J. (2014). Potential factors leading to the formation of cyanobacterial scums in a mesotrophic softwater lake in Ontario, Canada. Lake and Reservoir Management , 30 (4), 331-343.
Persaud, A. D., Paterson, A. M., Dillon, P. J., Winter, J. G., Palmer, M., & Somers, K. M. (2015). Forecasting cyanobacteria dominance in Canadian temperate lakes. Journal of Environmental Management , 151 , 343-352.
Peters, D. P., Laney, C. M., Lugo, A. E., Collins, S. L., Driscoll, C. T., Groffman, P. M., Grove, M., Knapp, A., Kratz, T. K., Ohman, M., Waide, R., & Yao, J. (2013). Long-term trends in ecological systems: a basis for understanding responses to global change . US Department of Agriculture, Agricultural Research Service. 378 p. https://www.fs.usda.gov/treesearch/pubs/46691
Pettersson, K., Grust, K., Weyhenmeyer, G., & Blenckner, T. (2003). Seasonality of chlorophyll and nutrients in Lake Erken–effects of weather conditions. Hydrobiologia , 506 , 75-81.
Pilla, R. M., Williamson, C. E., Zhang, J., Smyth, R. L., Lenters, J. D., Brentrup, J. A., ... & Fisher, T. J. (2018). Browning ‐ related decreases in water transparency l ead to long ‐ term increases in surface water temperature and thermal stratification in two small lakes. Journal of Geophysical Research: Biogeosciences , 123 (5), 1651-1665.
Prein, A. F., Rasmussen, R. M., Ikeda, K., Liu, C., Clark, M. P., & Holland, G. J. (2017). The future i ntensification of hourly precipitation extremes. Nature Climate Change , 7 (1), 48-52.
Prepas, E. E., & Vickery, J. (1984). Seasonal changes in total phosphorus and the role of internal loading i n Western Canadian lakes. Internationale Vereinigung für theoretische und angewandte Limnologie: Verhandlungen , 22 (1), 303-308.
R Core Team. (2021). R: A Language and Environment for Statistical Computing. R Foundation for Statistical Computing, Vienna, Austria. http://www.R-project.org/
Read, J. S., Hamilton, D. P., Desai, A. R., Rose, K. C., MacIntyre, S., Lenters, J. D., ... & Wu, C. H. (2012). Lake ‐ size dependency of wind shear and convection as controls on gas exchange. Geophysical Research Letters , 39 (9).
Reichwaldt, E. S., & Ghadouani, A. (2012). Effects of rainfall patterns on toxic cyanobacterial blooms in a changing climate: between simplistic scenarios and complex dynamics. Water Research , 46 (5), 1372-1393.
Reinl, K. L., Brookes, J. D., Carey, C. C., Harris, T. D., Ibelings, B., Morales-Williams, A. M., De Senerpont Domis, L. N., Atkins, K. S., Isles, P. D. F., Mesman, J. P., North, R. L., Rudstam, L. G., Stelzer, J. A. A., Venkiteswaran, J. J., Yokota, K., & Zhan, Q. (2021). Cyanobacterial blooms in oligotrophic lakes: Shifting the high-nutrient paradigm. Freshwater Biology , 66 (9), 1846-1859.
Reinl, K. L., Harris, T. D., North, R. L., Almela, P., Berger, S. A., Bizic, M., ... & Yokota, K. (2023). Blooms also like it cold. Limnology and Oceanography Letters .
Reynolds, C. S., Jaworski, G. H. M., Cmiech, H. A., & Leedale, G. F. (1981). On the annual cycle of the blue-green alga Microcystis aeruginosa Kütz. emend. Elenkin. Philosophical Transactions of the Royal Society of London. B, Biological Sciences , 293 (1068), 419-477.
Reynolds, C.S. (2006). The Ecology of Phytoplankton (Ecology, Biodiversity and Conservation) . Cambridge, UK: Cambridge University Press.
Richardson, D. C., Melles, S. J., Pilla, R. M., Hetherington, A. L., Knoll, L. B., Williamson, C. E., Kraemer, B. J., Jackson, J. R., Long, E. C., Moore, K., Rudstam, L. G., Rusak, J. A., Saros, J. E., Sharma, S., Strock, K. E., Weathers, K. C., & Wigdahl-Perry, C. R. (2017). Transparency, geomorphology and mixing regime explain variability in trends in lake temperature and stratification across Northeastern North America (1975–2014). Water , 9 (6), 442.
Rigosi, A., Carey, C. C., Ibelings, B. W., & Brookes, J. D. (2014). The interaction between climate warming and eutrophication to promote cyanobacteria is dependent on trophic state and varies among taxa. Limnology and Oceanography , 59 (1), 99-114.
Riley, E. T., & Prepas, E. E. (1984). Role of internal phosphorus loading in two shallow, productive lakes i n Alberta, Canada. Canadian Journal of Fisheries and Aquatic Sciences , 41 (6), 845-855.
Robarts, R. D., & Zohary, T. (1987). Temperature effects on photosynthetic capacity, respiration, and growth rates of bloom ‐ forming cyanobacteria. New Zealand Journal of Marine and Freshwater Research , 21 (3), 391-399.
Rohde, E., Pearce, N. J., Young, J., & Xenopoulos, M. A. (2022). Applying early warning indicators to predict critical transitions in a lake undergoing multiple changes. Ecological Applications , 32 (7), e2685.
Romo, S., Soria, J., Fernández, F., Ouahid, Y., & Barón ‐ Solá, Á. (2013). Water residence time and the dynamics of toxic cyanobacteria. Freshwater Biology , 58 (3), 513-522.
Rose, K. C., Winslow, L. A., Read, J. S., & Hansen, G. J. (2016). Climate ‐i nduced warming of lakes can be either amplified or suppressed by trends in water clarity. Limnology and Oceanography Letters , 1 (1), 44-53.
Reuter, W., & Müller, C. (1993). New trends in photobiology: adaptation of the photosynthetic apparatus of cyanobacteria to light and CO2. Journal of Photochemistry and Photobiology B: Biology , 21 (1), 3-27.
Sadro, S., Melack, J. M., Sickman, J. O., & Skeen, K. (2019). Climate warming response of mountain l akes affected by variations in snow. Limnology and Oceanography Letters , 4 (1), 9-17.
Scheffer, M., Carpenter, S., Foley, J. A., Folke, C., & Walker, B. (2001). Catastrophic shifts in ecosystems. Nature , 413 (6856), 591-596.
Scheffer, M., Bascompte, J., Brock, W. A., Brovkin, V., Carpenter, S. R., Dakos, V., Held, H., van Nes, E. H., Rietkerk, M., & Sugihara, G. (2009). Early-warning signals for critical transitions. Nature , 461 (7260), 53-59.
Scheffer, M., Carpenter, S. R., Dakos, V., & van Nes, E. H. (2015). Generic indicators of ecological resilience: inferring the chance of a critical transition. Annual Review of Ecology, Evolution, and Systematics , 46 , 145-167.
Schindler, D. W. (1977). Evolution of phosphorus limitation in lakes: natural mechanisms compensate for deficiencies of nitrogen and carbon in eutrophied lakes. Science , 195 (4275), 260-262.
Schindler, D. W. (2012). The dilemma of controlling cultural eutrophication of lakes. Proceedings of the Royal Society B: Biological Sciences , 279 (1746), 4322-4333.
Schindler, D. W., Carpenter, S. R., Chapra, S. C., Hecky, R. E., & Orihel, D. M. (2016). Reducing phosphorus to curb lake eutrophication is a success. Environmental Science & Technology , 50 (17), 8923-8929.
Schuurman, G. W., Hawkins Hoffman, C., Cole, D. N., Lawrence, D. J., Morton, J. M., Magness, D. R., Cravens, A. E., Covington, S., O’Malley, R., & Fisichelli, N. A. (2020). Resist-accept-direct (RAD)—a framework for the 21st-century natural resource manager. Natural Resource Report
NPS/NRSS/CCRP/NRR—2020/ 2213. National Park Service, Fort Collins, Colorado.
https://doi.org/10.36967/nrr-2283597.
Schuurman, G. (2021). Status of RAD Thinking in NPS. In: Climate Adaptation Using
Resist-Accept-Direct (RAD): Examples in Acadia National Park and Bandelier National Monument . Acadia National Park Science Symposium. https://www.youtube.com/watch?v=fjg4_d-gvj0
Seneviratne, S.I., Zhang, X., Adnan, M., Badi, W., Dereczynski, C., Di Luca, A., Ghosh, S., Iskandar, I., Kossin, J., Lewis, S., Otto, F., Pinto, I., Satoh, M., Vicente-Serrano, S. M., Wehner, M., & Zhou, B. (2021). Weather and Climate Extreme Events in a Changing Climate. In Climate Change 2021: The Physical Science Basis. Contribution of Working Group I to the Sixth Assessment Report of the Intergovernmental Panel on Climate Change [Masson-Delmotte, V., Zhai, P., Pirani, A., Connors, S. L., Péan, C., Berger, S., Caud, N., Chen, Y., Goldfarb, L., Gomis, M. I., Huang, M., Leitzell, K., Lonnoy, E., Matthews, J. B. R., Maycock, T. K., Waterfield, T., Yelekçi, O., Yu, R., Zhou, B. (eds.)]. Cambridge University Press, Cambridge, United Kingdom and New York, NY, USA, pp. 1513–1766, doi:10.1017/9781009157896.013.
Sharma, S., Gray, D. K., Read, J. S., O’Reilly, C. M., Schneider, P., Qudrat, A., ... & Woo, K. H. (2015). A global database of lake surface temperatures collected by in situ and satellite methods from 1985–2009. Scientific Data , 2 (1), 1-19.
Sirota, J., Baiser, B., Gotelli, N. J., & Ellison, A. M. (2013). Organic-matter loading determines regime shifts and alternative states in an aquatic ecosystem. Proceedings of the National Academy of Sciences , 110 (19), 7742-7747.
Smith, V. H., Tilman, G. D., & Nekola, J. C. (1999). Eutrophication: impacts of excess nutrient inputs on freshwater, marine, and terrestrial ecosystems. Environmental Pollution , 100 (1-3), 179-196.
Sorichetti, R. J., Creed, I. F., & Trick, C. G. (2014). The influence of iron, siderophores and refractory DOM on cyanobacterial biomass in oligotrophic lakes. Freshwater Biology , 59 (7), 1423-1436.
Spears, B. M., Futter, M. N., Jeppesen, E., Huser, B. J., Ives, S., Davidson, T. A., ... & Thackeray, S. J. (2017). Ecological resilience in lakes and the conjunction fallacy. Nature Ecology & Evolution , 1 (11), 1616-1624.
Stainsby, E. A., Winter, J. G., Jarjanazi, H., Paterson, A. M., Evans, D. O., & Young, J. D. (2011). Changes in the thermal stability of Lake Simcoe from 1980 to 2008. Journal of Great Lakes Research , 37 , 55-62.
Stoddard, J. L., Van Sickle, J., Herlihy, A. T., Brahney, J., Paulsen, S., Peck, D. V., Mitchell, R., & Pollard, A. I. (2016). Continental-scale increase in lake and stream phosphorus: are oligotrophic systems disappearing in the United States?. Environmental Science & Technology , 50 (7), 3409-3415.
Strock, K. E., Theodore, N., Gawley, W. G., Ellsworth, A. C., & Saros, J. E. (2017). Increasing dissolved organic carbon concentrations in northern boreal lakes: Implications for lake water transparency and thermal structure. Journal of Geophysical Research: Biogeosciences , 122 (5), 1022-1035.
Taranu, Z. E., Zurawell, R. W., Pick, F., & Gregory ‐ Eaves, I. (2012). Predicting cyanobacterial dynamics in the face of global change: the importance of scale and environmental context. Global Change Biology , 18 (12), 3477-3490.
Toffolon, M., Piccolroaz, S., Majone, B., Soja, A. M., Peeters, F., Schmid, M., & Wüest, A. (2014). Prediction of surface temperature in lakes with different morphology using air temperature. Limnology and Oceanography , 59 (6), 2185-2202.
Trimbee, A. M., & Harris, G. P. (1984). Phytoplankton population dynamics of a small reservoir: use of sedimentation traps to quantify the loss of diatoms and recruitment of summer bloom-forming blue-green algae. Journal of Plankton Research , 6 (5), 897-918.
Trimbee, A. M., & Prepas, E. E. (1988). The effect of oxygen depletion on the timing and magnitude of blue-green algal blooms: With 2 figures and 2 tables in the text. Internationale Vereinigung für theoretische und angewandte Limnologie: Verhandlungen , 23 (1), 220-226.
U.S. Environmental Protection Agency. (2000a). Nutrient Criteria Technical Guidance Manual, Lakes and Reservoirs. (1st Ed.). EPA-822-B00-001, April 2000.
U.S. Environmental Protection Agency. (2023, January 20). The Effects: Dead Zones and Harmful Algal Blooms. https://www.epa.gov/nutrientpollution/effects-dead-zones-and-harmful-algal-blooms
U.S. Environmental Protection Agency. (2023, September 28). Cyanobacterial Harmful Algal Blooms
(CyanoHABs) in Water Bodies. https://www.epa.gov/cyanohabs
Üveges, V., Tapolczai, K., Krienitz, L., & Padisák, J. (2012). Photosynthetic characteristics and physiological plasticity of an Aphanizomenon flos-aquae (Cyanobacteria, Nostocaceae) winter bloom in a deep oligo-mesotrophic lake (Lake Stechlin, Germany). Phytoplankton Responses to Human Impacts at Different Scales , 263-272.
Van Nes, E. H., & Scheffer, M. (2007). Slow recovery from perturbations as a generic indicator of a nearby catastrophic shift. The American Naturalist , 169 (6), 738-747.
Vermont Department of Health and Department of Environmental Conservation. (2023). Cyanobacteria (Blue-Green Algae) Tracker .
https://www.healthvermont.gov/environment/tracking/cyanobacteria-blue-green-algae-tracker
Visser, P. M., Ibelings, B. W., Bormans, M., & Huisman, J. (2016). Artificial mixing to control cyanobacterial blooms: a review. Aquatic Ecology , 50 , 423-441.
Vose, R.S., Easterling, D. R., Kunkel, K. E., LeGrande, A. N., & Wehner, M. F. (2017). Temperature changes in the United States. In: Climate Science Special Report: Fourth National Climate Assessment, Volume I [Wuebbles, D.J., Fahey, D. W., Hibbard, K. A., Dokken, D. J., Stewart, B. C., & Maycock, T. K. (eds.)]. U.S. Global Change Research Program, Washington, DC, USA, pp. 185-206, doi:
10.7930/J0N29V45.
Wagner, C., & Adrian, R. (2009). Cyanobacteria dominance: quantifying the effects of climate change. Limnology and Oceanography , 54 (6part2), 2460-2468.
Walsby, A. E. (2001). Determining the photosynthetic productivity of a stratified phytoplankton population. Aquatic Sciences , 63 , 18-43.
Warner, K. A., Fowler, R. A., Northington, R. M., Malik, H. I., McCue, J., & Saros, J. E. (2018). How does changing ice-out affect arctic versus boreal lakes? A comparison using two years with ice-out that differed by more than three weeks. Water , 10 (1), 78.
Warner, K. A., Fowler, R. A., & Saros, J. E. (2020). Differences in the effects of storms on dissolved organic carbon (DOC) in boreal lakes during an early summer storm and an autumn storm. Water , 12 (5), 1452
Warner, K. A., & Saros, J. E. (2019). Variable responses of dissolved organic carbon to precipitation events in boreal drinking water lakes. Water Research , 156 , 315-326.
Watson, S. B., Whitton, B. A., Higgins, S. N., Paerl, H. W., Brooks, B. W., & Wehr, J. D. (2015). Harmful Algal Blooms. In Freshwater Algae of North America (pp. 873-920). Academic Press.
Webster, K. E., Soranno, P. A., Cheruvelil, K. S., Bremigan, M. T., Downing, J. A., Vaux, P. D., ... & Connor, J. (2008). An empirical evaluation of the nutrient ‐ color paradigm for lakes. Limnology and Oceanography , 53 (3), 1137-1148.
Wehr, J. D., Sheath, R. G., & Kociolek, J. P. (Eds.). (2015). Freshwater Algae of North America: Ecology and Classification . Elsevier.
Wetzel, R. G. (2001), Limnology. San Diego: Academic Press, p. 151.
https://doi.org/10.1016/B978-0-08-057439-4.50001-0 ).
Weyhenmeyer, G. A. (2001). Warmer winters: are planktonic algal populations in Sweden's largest lakes affected?. AMBIO: A Journal of the Human Environment , 30 (8), 565-571.
Wiedner, C., Rücker, J., Brüggemann, R., & Nixdorf, B. (2007). Climate change affects timing and size of populations of an invasive cyanobacterium in temperate regions. Oecologia , 152 , 473-484.
Wight, P. (2019, September 19). Maine Lakes Expected To See More Intense, Frequent Toxic Algal Blooms Due To Climate Change . Maine Public.
https://www.mainepublic.org/environment-and-outdoors/2019-09-18/maine-lakes-expected-to-see-more-in tense-frequent-toxic-algal-blooms-due-to-climate-change
Wilkinson, G. M., Carpenter, S. R., Cole, J. J., Pace, M. L., Batt, R. D., Buelo, C. D., & Kurtzweil, J. T.
(2018). Early warning signals precede cyanobacterial blooms in multiple whole ‐l ake experiments. Ecological Monographs , 88 (2), 188-203.
Wilkinson, G. M., Walter, J. A., Buelo, C. D., & Pace, M. L. (2022). No evidence of widespread algal bloom intensification in hundreds of lakes. Frontiers i n Ecology and the Environment , 20 (1), 16-21.
Williamson, C. E., Brentrup, J. A., Zhang, J., Renwick, W. H., Hargreaves, B. R., Knoll, L. B., ... & Rose, K. C. (2014). Lakes as sensors in the landscape: Optical metrics as scalable sentinel responses to climate change. Limnology and Oceanography , 59 (3), 840-850.
Williamson, C. E., Overholt, E. P., Pilla, R. M., Leach, T. H., Brentrup, J. A., Knoll, L. B., Mette, E. M., & Moeller, R. E. (2015). Ecological consequences of long-term browning in lakes. Scientific Reports , 5 (1), 18666
Winslow, L., Read, J., Woolway, R., Brentrup, J., Leach, T., Zwart, J., Albers, S., & Collinge, D. (2019). rLakeAnalyzer: Lake physics tools. R package version 1.11.4.1.
https://CRAN.R-project.org/package=rLakeAnalyzer
Winter, J. G., DeSellas, A. M., Fletcher, R., Heintsch, L., Morley, A., Nakamoto, L., & Utsumi, K. (2011). Algal blooms in Ontario, Canada: increases in reports since 1994. Lake and Reservoir Management , 27 (2), 107-114.
Wong, S., & Hobbs, W. O. (2020). Exploring the Use of Fluorometric Sensors to Monitor Harmful Algal Blooms in Lakes . Publication 20-03-010. Washington State Department of Ecology, Olympia. https://apps. ecology.wa.gov/publications/SummaryPages/2003010.html.
Woolway, R. I., Kraemer, B. M., Lenters, J. D., Merchant, C. J., O’Reilly, C. M., & Sharma, S. (2020). Global lake responses to climate change. Nature Reviews Earth & Environment , 1 (8), 388-403.
Wu, X., Joyce, E. M., & Mason, T. J. (2011). The effects of ultrasound on cyanobacteria. Harmful Algae , 10 (6), 738-743.
Wurtsbaugh, W. A., Paerl, H. W., & Dodds, W. K. (2019). Nutrients, eutrophication and harmful algal blooms along the freshwater to marine continuum. Wiley Interdisciplinary Reviews: Water , 6 (5), e1373.
Wynne, T. T., Stumpf, R. P., Tomlinson, M. C., Schwab, D. J., Watabayashi, G. Y., & Christensen, J. D. (2011). Estimating cyanobacterial bloom transport by coupling remotely sensed imagery and a hydrodynamic model. Ecological Applications , 21 (7), 2709-2721.
Zhang, Y., Loiselle, S., Shi, K., Han, T., Zhang, M., Hu, M., Jing, Y., Lai, L., & Zhan, P. (2021). Wind effects for floating algae dynamics in eutrophic lakes. Remote Sensing , 13 (4), 800.
Zhang, Y., & Prepas, E. E. (1996). Regulation of the dominance of planktonic diatoms and cyanobacteria i n four eutrophic hardwater lakes by nutrients, water column stability, and temperature. Canadian Journal of Fisheries and Aquatic Sciences , 53 (3), 621-633.
Zhao, C. S., Shao, N. F., Yang, S. T., Ren, H., Ge, Y. R., Feng, P., Dong, B. E., & Zhao, Y. (2019). Predicting cyanobacteria bloom occurrence in lakes and reservoirs before blooms occur. Science of the Total Environment , 670 , 837-848.
Zhong, Y., Notaro, M., Vavrus, S. J., & Foster, M. J. (2016). Recent accelerated warming of the Laurentian Great Lakes: Physical drivers. Limnology and Oceanography , 61 (5), 1762-1786.