Part of a series of articles titled Reckoning with a Warming Climate.
Article
Crossing the Zero-Degree Threshold
RESEARCH REVIEW
Pamela J. Sousanes, Physical Scientist, Central Alaska Network and Arctic Network, National Park ServiceKen Hill, Physical Scientist, Central Alaska Network and Arctic Network, National Park Service
David Swanson, Terrestrial Ecologist, Arctic Network, National Park Service
Jonathan O’Donnell, Ecologist, Arctic Network, National Park Service
Peter Kirchner, Physical Scientist, Southwest Alaska Network, National Park Service
Deborah Kurtz, Natural Resource Program Manager, Kenai Fjords National Park, National Park Service
Michael Loso, Physical Scientist, Wrangell-St. Elias National Park and Preserve, National Park Service
Andrew Bliss, Physical Scientist, Southeast Alaska Network, National Park Service
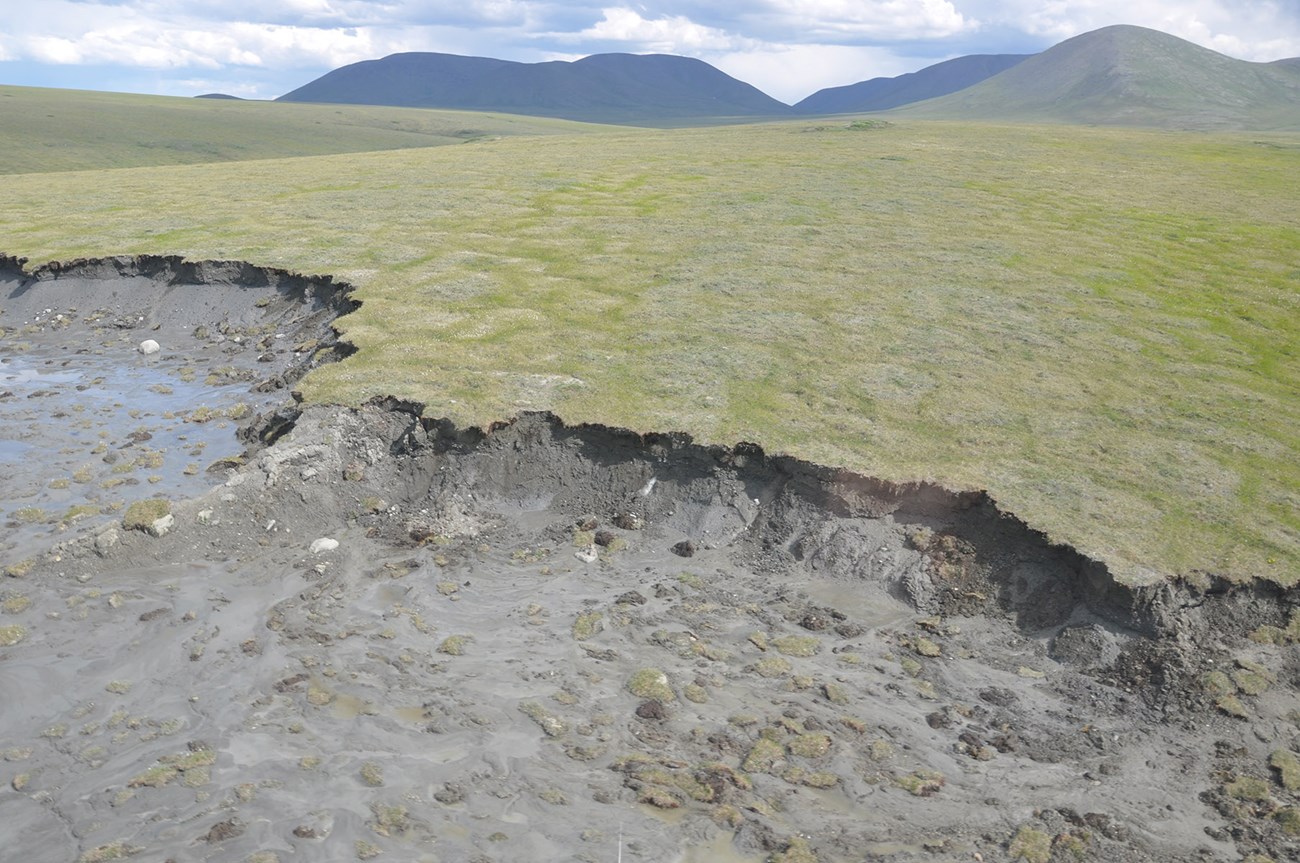
Alaska is among the fastest-warming regions on Earth. Recent studies show the rate of Arctic-wide warming is almost four times faster in the last four decades than anywhere else on the planet (Rantanen et al. 2022). Alaska national parks are undergoing change in response to disproportionate warming at high latitudes (Gonzalez et al. 2018). As the mean annual air temperature warms above the freezing threshold of 0°C, the frozen water, including glaciers, permafrost, and snow, will melt and thaw, altering the physical landscape. Here we provide a summary of key findings that focus on how even a small increase in temperature has a profound impact in Alaska parks that are defined by snow and ice.
Alaska’s Arctic Parks: Approaching Zero-Degree Celsius Threshold
The Arctic national parks have undergone tremendous climate variability over time, but the average temperature conditions we measure are now approaching, and in some cases crossing, the zero-degree threshold, which is unprecedented in modern times. Beyond this threshold, the cold conditions that define the Arctic and organize its landscapes and ecological processes cease, and the transformation to something we have no experience with begins.
Scientific studies in Arctic parks have documented the recent implications of climate change across the landscape as temperatures warm, permafrost thaws, and hydrologic pathways change. The northern regions of Alaska have warmed the most. This includes several parks in northwest Alaska, where the magnitude of temperature change in the past decade was two to three times greater than other parks in Alaska (Swanson et al. 2021). Climate models indicate that Earth’s higher latitudes, including Alaska, will continue to warm faster than other parts of the world (IPCC 2021, Lader et al. 2017). The presence of seasonal sea ice and snowpack historically characterized parks in the north and their unique cold climates. Temperatures in the Arctic parks are projected to warm by more than 7°C (on average) by the end of the century, and sea ice duration and seasonal snow cover will become less important defining features of the region (Littell 2023). Ballinger and Overland (2022) highlight the remarkable change that has occurred in Alaskan Arctic weather patterns over the last five years (2017-2021) with shifts in the Aleutian Low and polar jet stream funneling warmer southerly winds towards Alaska resulting in diminished sea ice growth. As warming trends continue, the Arctic will change.
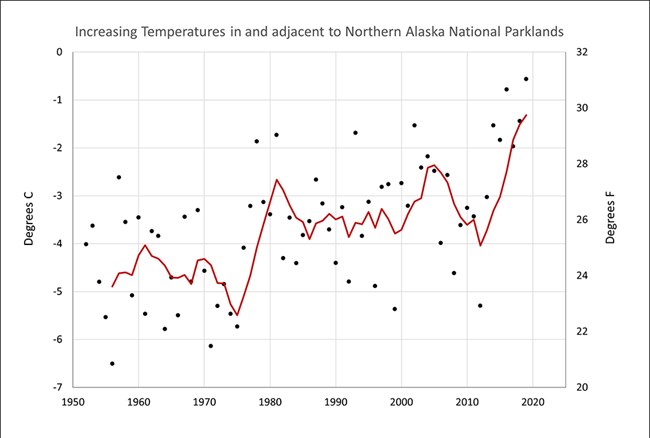
Notice the upward shift in temperatures in 2014. Also note that the latest increase puts annual temperatures near the 0°C freezing threshold.
The average annual air temperature of the Arctic parks has been below 0°C for tens of thousands of years. The cold temperature maintains permafrost (perennially frozen ground). Every degree of warming brings these frozen soils closer to thawing. Trends in Arctic Alaska’s average annual air temperatures in communities near the Arctic parks show a significant increase of more than 2.6°C between 1950 and 2021 (NOAA 2022, Ballinger et al. 2023). In the late 1970s Alaska’s statewide temperatures started to increase (Hartman and Wendler 2005). A more recent upward shift in temperatures occurred in 2014 and included the two warmest years on record for Alaska (Figure 1). We compared average annual air temperatures between the 2014 to 2019 period with the preceding 30-year period using weather stations in and around parks. We found that average air temperatures warmed by at least 1°C in most locations and up to 3°C in the northwest Arctic parks. During recent warm winters, the mean annual ground temperature at some of the warmer sites was above 0°C for the first time since we began measuring (Swanson et al. 2021).
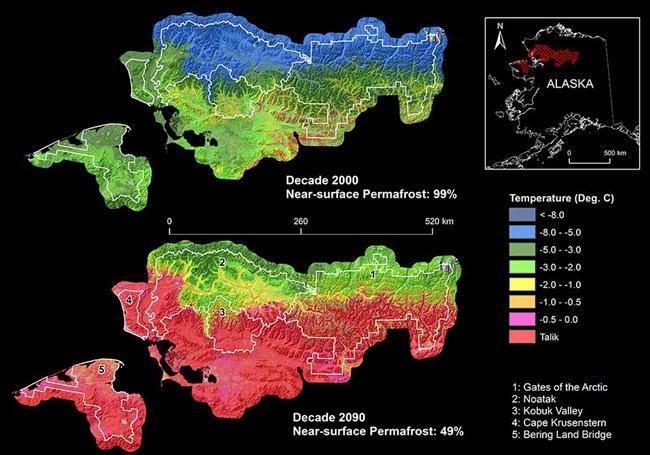
Data from the GIPL 1.0 permafrost model using climate data from CRU (2000s) and a composite of GCMs (2090s).
Permafrost is an important feature of northern and central Alaska, underlaying large swaths of the landscape (Jorgenson et al. 2008). Permafrost modeling studies indicated that at the start of the current century, permafrost underlaid nearly all of the five Arctic parks, but by the end of the century, about half of the permafrost in the Arctic parks will be thawing as a result of climate warming (Panda et al. 2014a, b, Panda et al. 2016; Figure 2). Thawing permafrost is one of the most impactful and widespread consequences of crossing the 0°C freezing threshold. Permafrost often contains masses of ice that melt when permafrost thaws, causing subsidence of the surface and sometime landslides.
Landscape-scale features that are perceptible on the surface are the easiest way to document change. However, the remoteness and sheer size of the northern Alaska parks makes it impossible to do this in person. Instead, images that are gathered from above, through satellites or aerial photography, enable scientists with a discerning eye to look for clues on the landscape that may indicate that the soils are warming. Swanson (2021a) used satellite images to locate numerous small landslides (active-layer detachments and retrogressive thaw slumps) across the Arctic parks that developed during the exceptionally warm summers in the mid-2000s. Swanson and Nolan (2018) used aerial photographs to document the continued growth of some of these thaw slumps in the following years.
Other features of these Arctic landscapes include frozen debris lobes, which are masses of frozen material that slowly creep downhill. When warmer temperatures penetrate deeper into these lobes, they destabilize the frozen material, allowing it to move downslope faster. Anything in the path of these debris lobes gets buried (Swanson 2021a). Frozen debris lobes that are just below 0°C are susceptible to run-away sliding with just a degree or two of warming.
Arctic lake drainage is another consequence of warming temperatures. Swanson (2019) found that Arctic coastal parks experienced a major episode of lake drainages following very warm years in 2005-2007 and 2018-2019. As the ground warms and ice melts, the ground surface subsides. When this happens along a lake shore, it opens new pathways for water to drain out of a lake. Deeper snowpacks and high-water levels during the spring melt can also erode the lake shore and carve new lake outlets. Once a new outlet opens, the lake might drain quickly, leaving behind a dry lakebed (Swanson 2019).
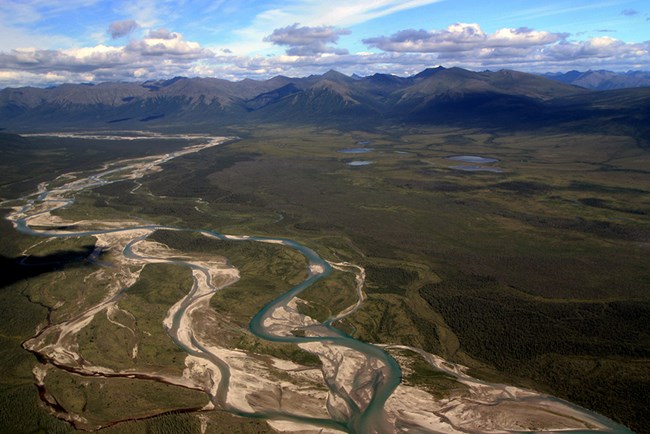
NPS/KEN HILL
Thawing permafrost also affects the hydro-logy of the northern landscape. Streamflow may be declining in headwater reaches due to the combined effects of vegetation change and permafrost thaw. Koch and others (2022) looked at eleven headwater catchments in northern Alaska parks that varied in forest cover and the degree of permafrost thaw. They identified two main climate change impacts to streamflow. First, climate change allows boreal treeline and shrub line expansion, which results in an increase in evapotranspiration, which is the physical and biological transport of water to the atmosphere. Second, permafrost thaw reroutes rainfall and soil water to deep groundwater aquifers, often bypassing the stream channel. Together, these processes can cause streamflow to decline and may contribute to channel drying. Headwater streams serve as critical habitat for native fish species in the Arctic, and this decline in flow may contribute to a loss of fish habitat (Koch et al. 2022).
Another surprising consequence of permafrost thaw in Arctic parks is that stream water may become cooler (Sjöberg et al. 2021). Model results from this study documented interesting hydrologic flow paths in Arctic systems related to ground thaw. Water that remained on top of continuous permafrost moved through shallow, warmer soil horizons enroute to the stream. In contrast, water that was not restricted by a frozen layer percolated down through unfrozen soils, flowing through deeper and cooler soil horizons, before entering the stream. The results of this study suggest that Arctic summer stream temperatures may decrease as the permafrost thaws and water finds its way through deeper cooler soils (Sjoberg et al. 2021), which can have direct effects on stream productivity and food webs.
Permafrost stores large amounts of organic carbon in frozen soil layers (Hugelius et al. 2014). As ground temperatures cross the zero-degree threshold and permafrost thaws, this carbon can be transformed and released to the atmosphere as the greenhouse gases carbon dioxide and methane (Schadel et al. 2016). Given the large amount of carbon stored in permafrost, its release to the atmosphere has the potential to further warm Earth’s climate (Schuur et al. 2013). As described above, permafrost thaw can also alter watershed hydrology and as a result, the transport of carbon from terrestrial to aquatic ecosystems. Not all permafrost soils in Alaska’s Arctic parks are the same. Soils vary in texture (silt, sand, gravel, etc.) and in the amount of ground ice within, including massive ice wedges. These soil properties affect groundwater movement and the forms of carbon that get transported to streams and rivers (O’Donnell et al. 2016). These observations suggest that as permafrost thaws, streams and rivers may have different chemical responses to this climate-induced disturbance (Toohey et al. 2016).
Most of the carbon stored in permafrost is very old, ranging from hundreds to thousands of years old (Estop-Aragones et al. 2020). One important question is how this old carbon will impact terrestrial and aquatic ecosystem function when it is released from thawing permafrost. O’Donnell and others (2020) tracked how old carbon was used by stream food webs in Alaska’s Arctic parks. They showed that resident fish, like Arctic grayling and Dolly Varden, take up old carbon when they eat stream invertebrates. Fish muscle tissue ranged in radiocarbon age from modern to more than 5,000 years before the present. More research is needed to understand how the use of old carbon affects stream food web function.
The timing of the snow season is closely coupled with air temperature, and as temperatures warm, the snow season is shorter in duration (Pan et al. 2020). A recent study found that Arctic summers are getting longer. Using images from remote cameras in Arctic parks to verify satellite data, one study found that the snow-free season has lengthened over the past 20 years. Vegetation is now greening up a week earlier in the spring and staying green a week later in the fall (Swanson 2021b).
The high-latitude parks in northern Alaska are the coldest in the nation but are at the point of profound change as temperatures warm, permafrost begins to thaw, and hydrologic pathways change. Warmer air temperatures are warming Arctic stream temperatures, causing lakes to drain as the permafrost beneath them melts, and are the catalyst for the release of carbon to the atmosphere and Arctic ecosystems as the ground surface thaws. When the average temperature of these places eventually climbs above freezing, it will be a very different Arctic.
A Climate Perspective from Northwest Alaska
Jeanette Koelsch shares her perspective on changes impacting her community and on lands within Bering Land Bridge National Preserve where she serves as the superintendent.
The changes to the land and the people are front and center for Bering Land Bridge National Preserve and the Western Arctic Parklands where changes in the timing and extent of sea ice and the ripple effects of a land that is losing its ice are impacting food resources, infrastructure, and livelihoods. Jeanette Koelsch is a tribal member of Nome Eskimo Community, the largest tribe in the Bering Strait Region. She shares her perspective on changes impacting her community and on lands within Bering Land Bridge National Preserve where she serves as the superintendent.
The people that live in and around national parks in Alaska are the most important resource. They have the firsthand practical knowledge of changes manifesting across the landscape, and they are also a vital component of that landscape. They are on the front line of climate changes and are acutely affected by what is happening.
Science and data are powerful tools when they are used in conjunction with local knowledge. Ongoing monitoring of weather, animal movements, hydrology, and vegetation patterns contribute to community knowledge. The information is integrated into regional and global analyses and climate models that are key to adaptation and mitigation planning efforts.
The following excerpts are used with permission from the University of Alaska Fairbanks (UAF) Project Jukebox Observing Change in Alaska's National Parks oral history interview conducted by Leslie McCartney, University of Alaska Fairbanks, and Katie Cullen, National Park Service in 2019 (McCartney 2019).
Jeanette Koelsch was born in Anchorage, Alaska to Joe and Grace Cross, and moved to Nome in 1982 when she was about ten years old. Her maternal grandparents were Jane and Jack Antoghame from St. Lawrence Island. From Jeanette’s perspective:
The continued land use for local people to ensure that lifestyle would continue [is important]. One of my main goals is to work with my staff to ensure those values are preserved … and creating stewards of not only the preserve, but everywhere.
When asked about how climate change impacts Bering Land Bridge, she says:
I see climate change as probably the number one threat to park resources. And to manage effectively, we have to be able to adapt to the change and do that in the most responsible way while still allowing for access and working with local people on subsistence.
One way park staff are interacting with local communities is through conversations:
Tribal consultation is something that’s taken very seriously here, and the needs and the inputs of the local people … And I see that trend in Alaska as we move forward.
The interview highlights some of the most significant changes from Jeanette’s personal perspective, starting with seasonal changes:
When I was a kid growing up here, winter was in September. By the time you went out to do moose hunt, there was usually hard ground and some snow… but now…the ground’s not frozen. [Early fall average temperatures have increased by 0.6°C in Nome (NOAA 2022a).]
Winter is the busy time for local people because the main access is by snowmachine. It is the time when locals do a lot of subsistence activities because the whole preserve is accessible.
Timing is difficult:
When I discuss changes with the local people it makes them sad, they are suffering from the unknown—of not knowing when to go and hunt something or if an animal will be there.
The shorter snow and ice season impacts access to food resources for coastal communities as well. For example, for Shismaref residents:
If the inlet doesn’t freeze, they have no access to the preserve, since they are on a barrier island. Even if there are moose, caribou, or musk ox, they can’t get to them.
Shorter snow and ice seasons limiting access is a thread running through most of the changes Jeanette described:
[Hunters are] … also going out farther to find ice to hunt marine mammals, more dangerous to go out five miles than one mile.
[Since 1980, the amount of sea ice that exists through summer has declined by >13% per decade and the ice that survives year-round is thinner and more fragile (NOAA 2022b).]
Impact of Powerful Autumn Storms on Coastal Western Alaska Communities
A very powerful early autumn storm slammed into the west coast of Alaska in September of 2022. The storm was notable because of the timing, intensity, and sheer size. Although, fall is generally the stormy season, in the past the presence of sea ice along the coast has buffered communities and protected them from powerful storm surges. In the case of remnant Typhoon Merbok, it was early in the season and there was no sea ice. This storm was fueled by anomalously warm water in the North Pacific Ocean southwest of Alaska. Storms usually fizzle when they move over the colder northern waters, but in this case, the warm water enabled it to keep its intensity.
This storm sheds light on how a single extreme event can be devasting for people who subsist off the land and take great care and time to prepare for a long, cold winter. Fish camps were destroyed, boats went missing, and freezers thawed along with a winter’s supply of food. Livelihoods were disrupted or upended by this event.
In a warmer world, with warmer oceans and longer ice-free seasons, Alaskan coastal com-munities will continue to be more vulnerable to intense fall storms. Koelsch adds:
From my observations, storms seem more frequent and stronger, action needs to happen to protect communities and subsistence camps. We need to work with communities and tribes to be more adaptive to climate change impacts.
McCartney, L. 2019.
Project Jukebox, Observing Change in Alaska’s National Parks, Oral History Interview with Jeanette Koelsch April17-18, 2019. Available at: https://jukebox.uaf.edu/p/3238 (accessed on September 7, 2022).
National Oceanic and Atmospheric Administration (NOAA) 2022a.
National Centers for Environmental Information, Climate at a Glance: City Time Series, Average Temperature, published August 2022. Available at: https://www.ncdc.noaa.gov/cag/ (accessed on September 7, 2022)
National Oceanic and Atmospheric Administration (NOAA). 2022b.
National Oceanic and Atmospheric Administration (NOAA) Climate.Gov, Science and Information for a Climate Smart Nation. September 2022. Available at: https://www.climate.gov (accessed on September 20, 2022)
Also See
Observing Change in Alaska’s National Parks
Capturing the effects of change on cultural and natural resources at these coastal parks, and hearing about the effects on the human connection to those resources allows audiences to gain first-hand understanding of a changing environment and provides the opportunity to draw comparisons between two distinct regions of Alaska.
Alaska’s Interior Parks: At the Zero-Degree Celsius Threshold
Parks in the central and southwest Alaska interior are in a more precarious position than the Arctic when it comes to nearing the 0°C threshold. A temperature increase of just a few degrees could alter the landscape. Many Interior Alaska parks have an annual average air temperature just below the freezing threshold. Temperatures increased abruptly in 2014 and many of the locations in and adjacent to Denali National Park and Preserve, Wrangell-St. Elias National Park and Preserve, and Yukon-Charley Rivers National Preserve experienced average annual temperatures at or above the freezing threshold of 0°C (Swanson et al. 2021). Permafrost extent maps show that about half of Denali and three-fourths of Wrangell-St. Elias had permafrost around the year 2000, but that it was susceptible to thaw because ground temperatures were at or just below 0°C (Panda et al. 2014a, b). Modeling by Panda and others (2014a, b) predict that most of the permafrost in these two parks will start to thaw by the end of the current century. The implications of thawing permafrost are more immediate and widespread in this region. Large swaths of parklands and communities are at the precipice of melt, with immediate and visible changes to the physical landscape and costly impacts to park infrastructure.
Permafrost landscapes in Interior Alaska parks are already experiencing dramatic changes in response to climate, ecological succession, and fire (Jorgenson et al. 2020). Fire history, soil properties, hydrology, air temperature, and snow cover all interact and affect ground temperatures and the potential for permafrost degradation in boreal peatlands which are common throughout Interior Alaska. Long-term monitoring at Gosling Lake, near Lake Minchumina in Denali National Park and Preserve, showed increasing permafrost degradation in recent decades in response to long-term warming, recent warm and wet summers, and low-severity fire (Jorgenson 2022). However, short-term variability also plays a key role. Snow acts as a blanket, insulating the permafrost from frigid winter air temperatures. Extreme seasonal weather with cold winters and little snow resulted in the temporary recovery of permafrost in some bog landscapes. This shows that when ground temperatures are near zero, relatively small changes in temperature can result in large landscape changes.
Climate warming is thawing permafrost, which can weaken earth materials and increase the occurrence of landslides (Patton et al. 2019, Lader et al. 2023). There are numerous landslides in the vicinity of the Denali Park Road that were initiated or aggravated by permafrost thaw, leading to road closures and costly repairs (Capps et al. 2017, Patton et al. 2020, 2021). Patton and others (2021) looked at three shallow-angle, thaw-initiated landslides in Denali to quantify the deformation and movement. The authors found that there was no evidence of shallow permafrost within a recent landslide, indicating that when the surface was disturbed there was an increase in the amount of heat available to thaw soils below the surface. This is an example of a positive feedback loop where warming temperatures lead to an acceleration of permafrost thaw in recent landslides (Patton et al. 2021).
Snow cover plays an important role on the landscape; it regulates local and regional temperatures by reflecting incoming solar radiation and controlling evaporation, condensation, and sublimation of water. Snow cover also protects vegetation and wildlife that lives under the snow from sub-zero air temperatures and opens the landscape to snow travel for transportation and subsistence activities. When temperatures exceed 0°C, precipitation falls more frequently as rain rather than snow, which can impact wildlife, plants, and people. This temperature and rain-versus-snow threshold also dictates the duration of the snow cover season, with warming temperatures changing the timing (phenology) of the seasons.
A recent study showed that the amount of sunlight that is reflected by the ground (known as albedo) declined by 79% when dry winter snow melted in the spring (Kim et al. 2018). Along the way from snow to no-snow, the study found that when the snowpack starts to thaw and refreeze each day in the spring, there is a 25% decrease in snow cover albedo in Alaska. As albedo goes down, the surface absorbs more energy from the sun, reinforcing snowmelt and surface warming. This contributes to an earlier growing season onset and activation of seasonal biological and hydrological processes (Kim et al. 2018).
As temperatures increase and influence other processes like snow melt and albedo, the number of snow-free days is increasing. Earlier snow-off dates (date when the seasonal snowpack melts in the spring) lead to longer growing seasons, permafrost degradation, changes in hydrology, and a host of other ecosystem processes. Using remote sensing, Pan et al. 2020, 2021 found a trend toward earlier snow-off timing from 1988-2018 across Alaska. The average trend was 0.39 days per year or 12 days over the study period, but the trend varied from 0.11 to 1.31 days per year depending on the location. Snow-off was markedly earlier in warm years and more variable in recent years.
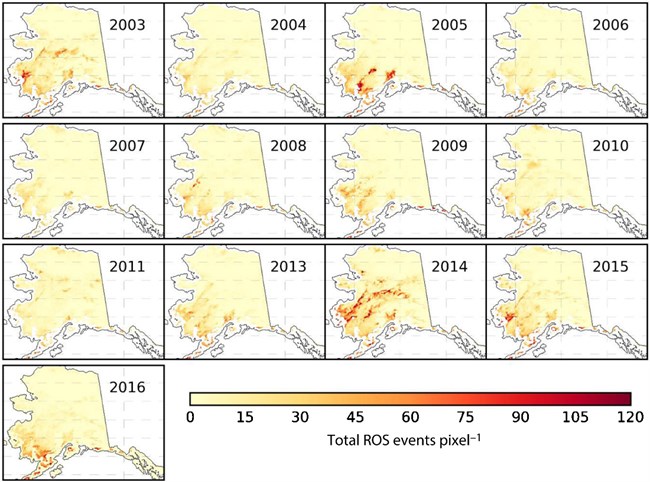
Rain-on-snow (ROS), or “wet” snow events affect the hydrology, wildlife, and human activity in and around Alaska’s parks. ROS events can influence caribou movement during the winter season (Loe et al. 2016) and reduce caribou’s access to forage under the snow. The rain freezes on top of the snow creating a hard crust and a barrier to the lichens on the ground below. Projections indicate an increased likelihood of ROS events across caribou ranges in northern Alaska (Bieniek et al. 2018). ROS events are most common during autumn and spring months along the maritime Bering Sea coast and boreal interior regions but are infrequent on the colder Arctic North Slope. Their frequency and extent coincide with greater climate variability and warmer temperatures regionally (Figure 3). While further study of ROS is needed, initial modeling results suggest that as high-latitude temperatures increase, wet snow events will also increase in frequency and extent, particularly in the southwestern and interior regions of Alaska (Pan et al. 2018a, b).
Littell and others (2018) looked at the statewide snow response to climate change using historical data and global climate models. As more locations in Alaska transition across the 0 °C threshold, the number of months with reliable snow cover decreases, mainly at lower elevations and lower latitudes. Littell (2023) recently mapped climate change and impact projections for the national parks in Alaska. The future scenarios for snowfall included both a mid- and late-century time frame, and both a medium and high range of warming. In all scenarios and at all parks temperatures warmed and total annual precipitation increased. The snowfall trajectories vary by location and depend on the annual temperature of the region. Higher latitudes and higher elevations will stay colder longer, and the increase in winter precipitation will be in the form of snow.
Alaska’s Coastal Mountain Parks: Straddling the Zero-Degree Celsius Threshold
Alaska parks along the southern coast have the largest proportion of glaciers and ice fields of any in the country—they are high enough in latitude and have high-elevation mountain ranges that capture moisture spinning off the Pacific Ocean as snow. Glaciers and glacier systems are the dominant physical features in these parks and are inextricably linked to climate fluctuations.
Delineation of glacier-covered area in the state of Alaska indicates overall glacier-covered area decreased by 13% (3,253 square miles or 8,425 km2) between 1985 and 2020 (Roberts-Pierel et al. 2021). During the same period supraglacial debris expanded by 64% (1,081 square miles or 2,799 km2) suggesting a significant loss in volume as well. This study also shows a clear and ongoing trend of reduced area, especially at the mid and lower elevations, where the 0-degree isotherm is changing most rapidly. Furthermore, an earlier study showed the glacier-covered area in the Alaskan parks decreased by 8% between the 1950s and the early 2000s suggesting that the rate of loss is also increasing (Loso et al. 2014).
As temperatures warm and glaciers retreat, what was recently covered in ice becomes newly exposed land or water with implications for surface albedo, ecosystems and downstream hydrologic conditions. A recent study in Kenai Fjords National Park documented rapid maritime glacier retreat and terminus change at 19 glaciers between 1984-2021 that resulted in a cumulative loss of 16 square miles (42 km2) of ice (Black and Kurtz 2022; Figure 4). As glaciers melt, new habitats form in their wake. In some cases, new recreation opportunities develop, too, such as at the expanded proglacial lake at Kenai Fjords’ Bear Glacier where visitation has increased in the past decade.
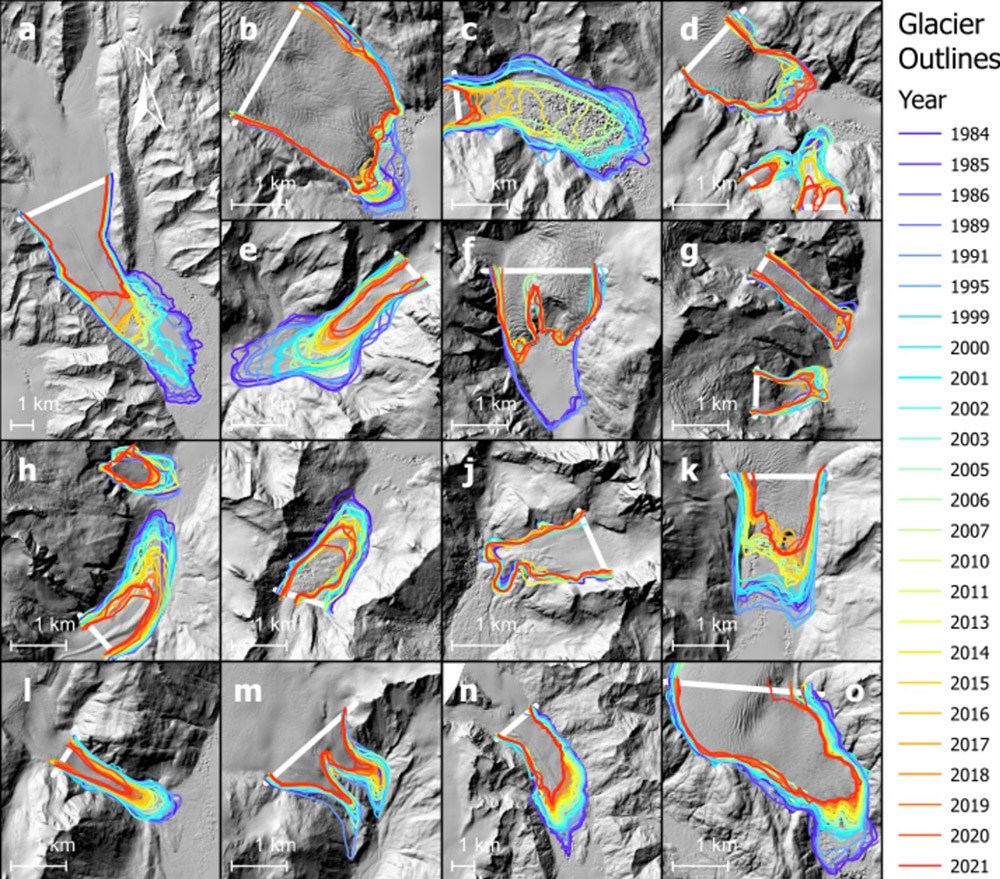
The color scale ranges from purple as the oldest (1984) to red as the youngest (2021). The base image is a hillshade of a DEM from a U.S. Fish and Wildlife Service-led structure-from-motion data acquisition in 2016. From Black and Kurtz 2022.
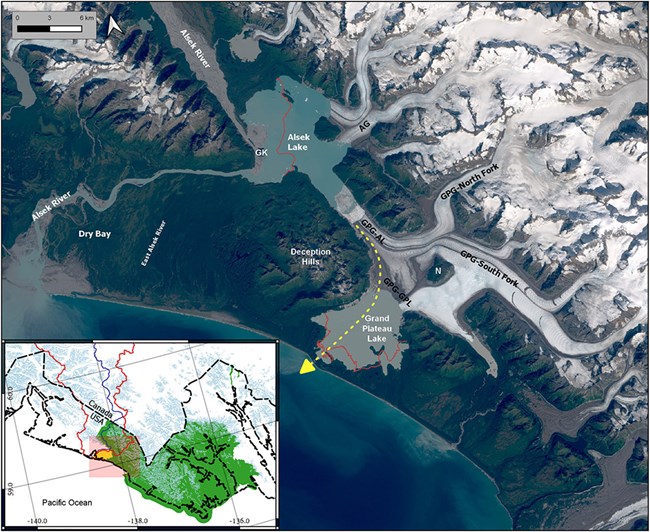
Green polygon is Glacier Bay National Park, and the yellow polygon is Glacier Bay National Preserve, centered over the lower Alsek River and Dry Bay. Base is Sentinel-2 image acquired September 10, 2018; Albers equal area projection. From Loso et al. 2021
Recent monitoring efforts in Glacier Bay National Park and Preserve found that, in some cases, glacier retreat can reroute the outlet of a major river, resulting in not only ecological impacts, but significant socioeconomic impacts as well. Loso and others (2021) predict that due to thinning glacier termini, the Alsek River will abandon its present Dry Bay outlet channel in favor of the much steeper outlet of Grand Plateau Lake, more than 17 miles (28 km) to the southeast in the federally designated wilderness of Glacier Bay National Park (Figure 5). Traditional and modern human activities dependent on the Alsek River in Dry Bay include commercial fishing, subsistence and sport hunting and fishing, and world-renowned wilderness rafting expeditions.
The Phase Shift
Alaska parks are in a state of change as the frozen components of the landscapes warm. As average annual temperatures climb above 0 °C, permafrost has begun to thaw and, with this phase shift, there has been a myriad of changes. Thawing ground increases landslide risks and erosion along park roads and rivers and wreaks havoc on infrastructure that has been designed for frozen soils. Warming temperatures are affecting snow cover, increasing the length of the snow-free season, decreasing surface albedo and increasing the frequency of rain-on-snow or wet snow events with cascading impacts to park resources. Glaciers, while retaining snow up high, are receding and thinning at lower elevations and this has significant impacts for subsistence, park management, and recreational pursuits in the parks. This critical phase shift from frozen to unfrozen is happening now and will continue into the future, impacting all Alaska parks.
References
Ballinger, T. J. and J. E. Overland. 2022.
The Alaskan Arctic regime shift since 2017: A harbinger of years to come? Polar Science 32: 100841.
Bieniek, P. A., U. S. Bhatt, J. E. Walsh, R. Lader, B. Griffith, J. K. Roach, and R. L. Thoman, 2018.
Assessment of Alaska rain-on-snow events using dynamical downscaling. J. Appl. Meteor. Climatol. 57: 1847-1863.
Black, T. and D. Kurtz. 2022.
Maritime glacier retreat and terminus area change in Kenai Fjords National Park, Alaska, between 1984 and 2021. Journal of Glaciology 1-15.
Boelman, N. T., G. E. Liston, E. Gurarie, A. J. H. Meddens, P. J. Mahoney, P. B. Kirchner, G. Bohrer, T. J. Brinkman, C. L. Cosgrove, J. U. H. Eitel, M. Hebblewhite, J. S. Kimball, S. LaPoint, A. W. Nolin, S. H. Pedersen, L. R. Prugh, A. K. Reinking, and L. A. Vierling. 2019.
Integrating snow science and wildlife ecology in Arctic-boreal North America. Environmental Research Letters 14(1): 010401.
Gonzalez, P., F. Wang, M. Notaro, D. J. Vimont, and J. W. Williams. 2018.
Disproportionate magnitude of climate change in United States national parks. Environmental Research Letters 13(10): 104001.
Estop-Aragonés, C., D. Olefeldt, B. W. Abbott, J. P. Chanton, C. I. Czimczik, J. F. Dean, J. E. Egan, L. Gandois, M. H. Garnett, I. P. Hartley, A. Hoyt, M. Lupascu, S. M. Natali, J. A. O’Donnell, P. A. Raymond, A. J. Tanentzap, S. E. Tank, E. A. G. Schuur, M. Turetsky, and K. Walter Anthony. 2020.
Assessing the potential for mobilization of old soil carbon after permafrost thaw: A synthesis of 14C measurements from the northern permafrost region. Global Biogeochemical Cycles 34(9): e2020GB006672.
Ewing, S. A., J. A. O’Donnell, G. R. Aiken, K. Butler, D. Butman, L. Windham-Myers, and M. Z. Kanevskiy. 2015.
Long-term anoxia and release of ancient, labile carbon upon thaw of Pleistocene permafrost. Geophysical Research Letters 42(24): 10,730-10,738.
Hartman, B. and G. Wendler. 2005.
The significance of the 1976 Pacific climate shift in the climatology of Alaska. Journal of Climate, 18 (22): 4824-4839.
Hugelius, G., J. Strauss, S. Zubrzycki, J. W. Harden, E. A. G. Schuur, C.-L. Ping, L. Schirrmeister, G. Grosse, G. J. Michaelson, C. D. Koven, J. A. O’Donnell, B. Elberling, U. Mishra, P. Camill, Z. Yu, J. Palmtag, and P. Kuhry. 2014.
Estimated stocks of circumpolar permafrost carbon with quantified uncertainty ranges and identified data gaps. Biogeosciences 11 (23): 6573-6593.
Intergovernmental Panel on Climate Change (IPCC). 2021.
[Masson-Delmotte, V., P. Zhai, A. Pirani, S.L. Connors, C. Péan, S. Berger, N. Caud, Y. Chen, L. Goldfarb, M.I. Gomis, M. Huang, K. Leitzell, E. Lonnoy, J.B.R. Matthews, T.K. Maycock, T. Waterfield, O. Yelekçi, R. Yu, and B. Zhou (eds.)]
Climate Change 2021: The Physical Science Basis. Contribution of Working Group I to the Sixth Assessment Report of the Intergovernmental Panel on Climate Change Cambridge University Press, Cambridge, United Kingdom and New York, NY, USA.
Jorgenson, M. Torre, Thomas A. Douglas, Anna K. Liljedahl, Joanna E. Roth, Tim C. Cater, Wendy A. Davis, Gerald V. Frost, Patricia F. Miller, and Charles H. Racine. 2020.
The roles of climate extremes, ecological succession, and hydrology in repeated permafrost aggradation and degradation in fens on the Tanana Flats, Alaska. Journal of Geophysical Research: Biogeosciences 125 (12): e2020JG005824.
Jorgenson, M.T., M. Kanevskiy, C. Roland, K. Hill, D. Schirokaer, S. Stehn, B. Schroeder, and Y, Shur. 2022.
Repeated permafrost formation and degradation in boreal peatland ecosystems in relation to climate extremes, fire, ecological shifts, and a Geomorphic legacy. Atmosphere 13: 1170.
Kim, Y., J. Kimball, J. Du, C. Schaaf, and P. Kirchner. 2018.
Quantifying the effects of freeze-thaw transitions and snowpack melt on land surface albedo and energy exchange over Alaska and Western Canada. Environmental Research Letters 13: 075009.
Koch, J. C. Y. Sjoberg, J. O’Donnell, M. Carey, P. F. Sullivan and A. Terskaia. 2022.
Sensitivity of headwater streamflow to thawing permafrost and vegetation change in a warming Arctic. Environmental Research Letters 17(4): 044074.
Lader, R., J. E. Walsh, U. S. Bhatt, and P. A. Bieniek. 2017.
Projections of twenty-first-century climate extremes for Alaska via dynamical downscaling and quantile mapping. Journal of Applied Meteorology and Climatology 56(9): 2393-2409.
Lader, R. J., P. Sousanes, U. S. Bhatt, J. E. Walsh, and P. A. Bieniek. 2023.
Climate Indicators of landslide risks on Alaska national park road corridors. Atmosphere 14 (1).
Littell, J., S. McAfee, and G. Hayward. 2018.
Alaska snowpack response to climate change: Statewide snowfall equivalent and snowpack water scenarios. Water 10(5): 668.
Littell, J. S. 2023.
Alaska climate futures (mid and late 21st century) and historical references (20th century): U.S. Geological Survey Data Release.
Loe, L.E., B.B. Hansen, A. Stien, S.D. Albon, R. Bischof, A. Carlsson, R.J. Irvine, M. Meland, I.M. Rivrud, E. Ropstad, V. Veiberg and A. Mysterud. 2016.
Behavioral buffering of extreme weather events in a high-Arctic herbivore. 2016. Ecosphere 7(6): e01374.
Loso, M. G., C. F. Larsen, B. S. Tober, M. Christoffersen, M. Fahnestock, J. W. Holt, and M. Truffer. 2021.
Quo vadis, Alsek? Climate-driven glacier retreat may change the course of a major river outlet in southern Alaska. Geomorphology 384: 107701.
Markon, C., S. Gray, M. Berman, L. Eerkes-Medrano, T. Hennessy, H. P. Huntington, J. S. Littell, M. McCammon, R. Thoman, and S. Trainor. 2018.
Alaska. pp. 1185–1241 In Impacts, Risks, and Adaptation in the United States: Fourth National Climate Assessment, Volume II. Washington, D.C.: U.S. Global Change Research Program.
NOAA Regional Climate Centers. 2022.
XmACIS2.
O’Donnell, J. A., G. R. Aiken, D. K. Swanson, S. Panda, A. P. Baltensperger, and K. D. Butler. 2017.
Dissolved organic matter composition of Arctic rivers: Linking permafrost and parent material to riverine carbon. Global Biogeochemical Cycles 30 (12): 1811-1826.
O’Donnell, J. A., M. P. Carey, J. C. Koch, X. Xu, B. A. Poulin, J. Walker, and C. E. Zimmerman. 2020.
Permafrost hydrology drives the assimilation of old carbon by stream food webs in the Arctic. Ecosystems 23(2): 435-453.
Pan, C. G., P. B. Kirchner, J. S. Kimball, J. Du, and M. A. Rawlins. 2021.
Snow phenology and hydrologic timing in the Yukon River Basin, AK, USA. Remote Sensing 13(12): 2284.
Pan, C. G., P. B. Kirchner, J. S. Kimball, and J. Du. 2020.
A long-term passive microwave snowoff record for the Alaska region, 1988-2016. Remote Sensing 12(1): 153.
Pan, C. G., P. B. Kirchner, J. S. Kimball, Y. Kim, and J. Du. 2018a.
Rain-on-snow events in Alaska, their frequency and distribution from satellite observations. Environmental Research Letters 13(7): 075004.
Pan, C. G., P. B. Kirchner, J. S. Kimball, Y. Kim, and J. Du. 2018b.
ABoVE: Rain-on-snow frequency and distribution during cold seasons, Alaska, 2003-2016. ORNL DAAC, Oak Ridge, Tennessee, USA.
Panda, S. K., S. S. Marchenko, and V. E. Romanovsky. 2014a.
High-resolution permafrost modeling in Denali National Park and Preserve. Natural Resource Report NPS/CAKN/NRTR—2014/858. Fort Collins, Colorado: National Park Service.
Panda, S. K., S. S. Marchenko, and V. E. Romanovsky. 2014b.
High-resolution permafrost modeling in Wrangell-St. Elias National Park and Preserve. Natural Resource Report NPS/CAKN/NRTR—2014/861. Fort Collins, Colorado: National Park Service.
Panda, S. K., S. S. Marchenko, and V. E. Romanovsky. 2016.
High-resolution permafrost modeling in the Arctic Network of national parks, preserves and monuments. Natural Resource Report NPS/ARCN/NRR-2016/1366. Fort Collins, Colorado: National Park Service.
Patton, A. I., S. L. Rathburn, D. M. Capps, D. McGrath, and R. A. Brown. 2021.
Ongoing landslide deformation in thawing permafrost. Geophysical Research Letters 48(16): e2021GL092959.
Rantanen, M., A. Y. Karpechko, A. Lipponen, K. Nordling, O. Hyvärinen, K. Ruosteenoja, T. Vihma, and A. Laaksonen. 2022.
The Arctic has warmed nearly four times faster than the globe since 1979. Communications Earth & Environment 3(1): 168.
Roberts-Pierel, B. M., P. B. Kirchner, J. B. Kilbride, and R. E. Kennedy. 2022.
Changes over the last 35 years in Alaska’s glaciated landscape: A novel deep learning approach to mapping glaciers at fine temporal granularity. Remote Sensing 14(18): 4582.
Schuur, E. A. G., B. W. Abbott, W. B. Bowden, V. Brovkin, P. Camill, J. G. Canadell, J. P. Chanton, F. S. Chapin III, T. R. Christensen, P. Ciais, B. T. Crosby, C. I. Czimczik, G. Grosse, J. Harden, D. J. Hayes, G. Hugelius, J. D. Jastrow, J. B. Jones, T. Kleinen, C. D. Koven, G. Krinner, P. Kuhry, D. M. Lawrence, A. D. McGuire, S. M. Natali, J. A. O’Donnell, C. L. Ping, W. J. Riley, A. Rinke, V. E. Romanovsky, A. B. K. Sannel, C. Schädel, K. Schaefer, J. Sky, Z. M. Subin, C. Tarnocai, M. R. Turetsky, M. P. Waldrop, K. M. Walter Anthony, K. P. Wickland, C. J. Wilson, and S. A. Zimov. 2013.
Expert assessment of vulnerability of permafrost carbon to climate change. Climatic Change 119(2): 359-74.
Schädel, C., M. K. F. Bader, E. A. G. Schuur, C. Biasi, R. Bracho, P. Čapek, S. De Baets, K. Diáková, J. Ernakovich, C. Estop-Aragones, D. E. Graham, I. P. Hartley, C. M. Iversen, E. Kane, C. Knoblauch, M. Lupascu, P. J. Martikainen, S. M. Natali, R. J. Norby, J. A. O’Donnell, T. Roy Chowdhury, H. Šantrůčková, G. Shaver, V. L. Sloan, C. C. Treat, M. R. Turetsky, M. P. Waldrop, and K. P. Wickland. 2016.
Potential carbon emissions dominated by carbon dioxide from thawed permafrost soils. Nature Climate Change 6(10): 950-53.
Swanson, D. K., P. J. Sousanes, and K. Hill. 2021.
Increased mean annual temperatures in 2014-2019 indicate permafrost thaw in Alaskan national parks. Arctic, Antarctic, and Alpine Research 53(1): 1-19.
Swanson, D. K. and M. Nolan. 2018.
Growth of retrogressive thaw slumps in the Noatak Valley, Alaska, 2010-2016, measured by airborne photogrammetry. Remote Sensing 10(7): 983.
Swanson, D. K. 2019.
Thermokarst and precipitation drive changes in the area of lakes and ponds in the National Parks of northwestern Alaska, 1984-2018. Arctic, Antarctic, and Alpine Research 51(1): 265-279.
Swanson, D. K. 2021a.
Permafrost thaw-related slope failures in Alaska’s Arctic National Parks, c. 1980-2019. Permafrost and Periglacial Processes 32(3): 392-406.
Swanson, D. K. 2021b.
Vegetation and snow phenology monitoring in the Arctic Network through 2020: Results from satellites and land-based cameras. Natural Resource Report NPS/ARCN/NRR—2021/2337. Fort Collins, Colorado: National Park Service.
Sjöberg, Y., A. Jan, S. L. Painter, E. T. Coon, M. P. Carey, J. A. O’Donnell, and J. C. Koch. 2021.
Permafrost promotes shallow groundwater flow and warmer headwater streams. Water Resources Research 57(2): e2020WR027463.
Toohey, R. C., N. M. Herman-Mercer, P. F. Schuster, E. A. Mutter, and J. C. Koch. 2016.
Multidecadal increases in the Yukon River basin of chemical fluxes as indicators of changing flowpaths, groundwater, and permafrost. Geophysical Research Letters 43 (23): 12,120-12,130.
Last updated: November 9, 2023