Part of a series of articles titled Alaska Park Science Volume 20 Issue 1 - Parks as Proving Grounds.
Article
New Approaches to Study Interactions Among Climate, Environment, and Humans in Arctic Alaska
Karen J. Wang, Brown University
Jonathan A. O’Donnell, National Park Service
Yongsong Huang, Brown University
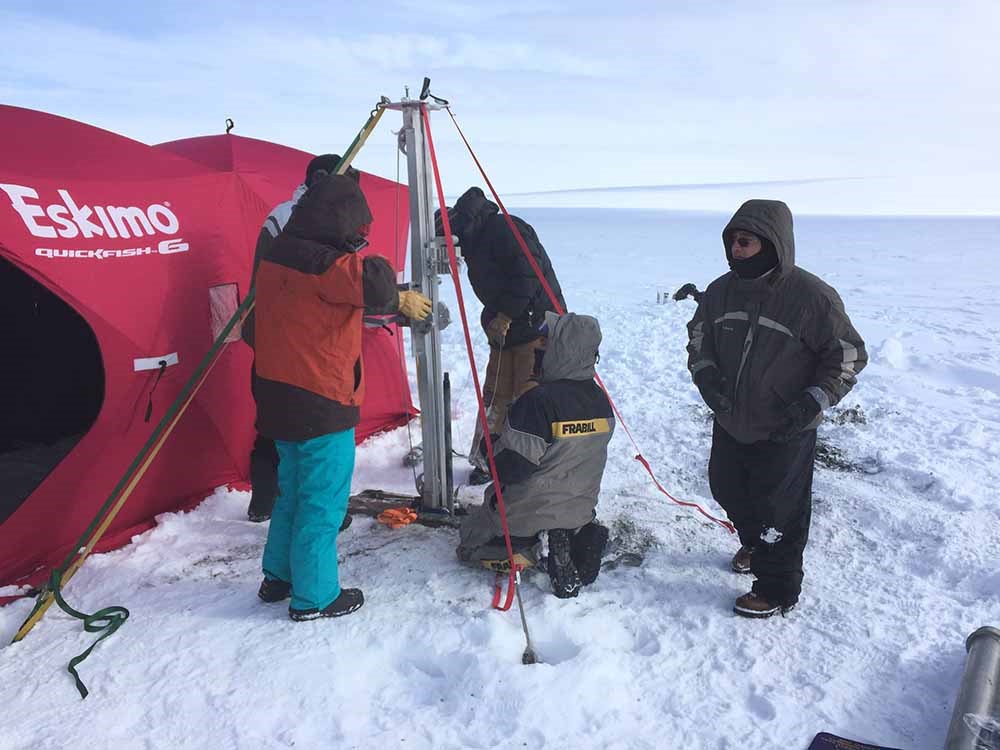
Skydance Aviation/Scott Amy
The Arctic is rapidly changing, with air temperatures rising nearly two times faster than those of lower latitudes over the past 150 years (Bekryaev et al. 2010). Rapid and pronounced warming in the Arctic is caused not only by rising emissions of greenhouse gases from human activities (fossil fuel burning, land use change), but also by Arctic amplification (Serreze and Barry 2011), a phenomenon by which the magnitude of climate change occurs more strongly in the poles. Alaska and its national parks are among regions bearing the brunt of climate change’s effects (Gonzalez et al. 2018). The negative consequences of climate change include permafrost thaw, sea ice loss, more severe wildfires, coastal erosion, changing habitat (including human habitats), and animal and plant species distributions. But how do we know that the recent climate change in the Arctic is exceptional and outside the natural range of climate variability? What are the sensitivities of various landscape processes to changes in temperatures and what does this tell us about how the Arctic will change in the future? Geological records like lake and ocean sediments can help us understand how various factors (e.g., greenhouse gases, solar insolation) have driven these changes in the past, which therefore allow us to make more informed predictions of future changes.
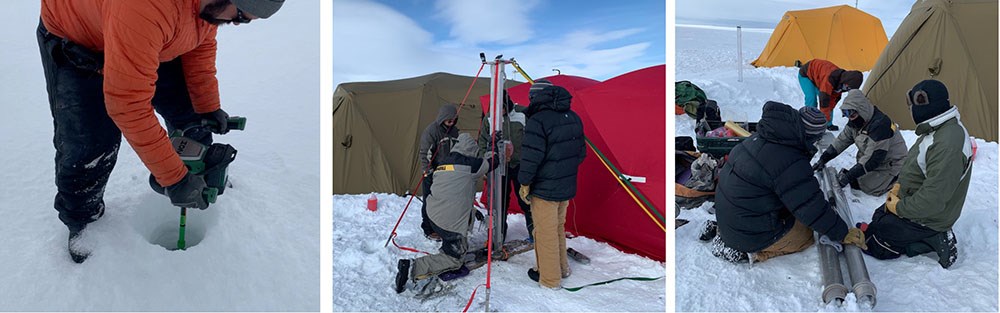
Skydance Aviation/Scott Amy
Lake sediments accumulate for thousands to hundreds of thousands of years, recording environmental archives that can provide context for long-term climate and ecological variabilities as well as short-term observational records. In this way, understanding the Arctic’s past is a key to understanding and providing context for what we see today, and also for predicting what we can expect for the future. As paleoclimatologists and paleoecologists, we can deduce environmental changes of the past by examining lake sediment cores. By using a piston coring system afloat a raft or atop of ice (Figure 1), we can extract sediment cores from lakebeds. The techniques we use to date sediment horizons depend on the age of the sediment (Figure 2). For example, for sediments deposited within the last 200 years or so, we can determine ages by measuring the lead isotope (210Pb) content of sediments (Binford 1990). For sediments deposited within the last 45,000 years, we can assign ages by measuring the radiocarbon (14C) content of organic matter preserved in sediment layers. To date sediments beyond 45,000 years in age, we can use paleomagnetics, thermoluminescence, and tephra (ash layers ejected by local and far-off volcanic eruptions) deposits of known ages. After determining the age of the sediments as a function of depth, we analyze the fossils and chemicals from past plants and animals that are stratigraphically archived and available to understand the nature and timing of environmental changes of the past (Figure 2).
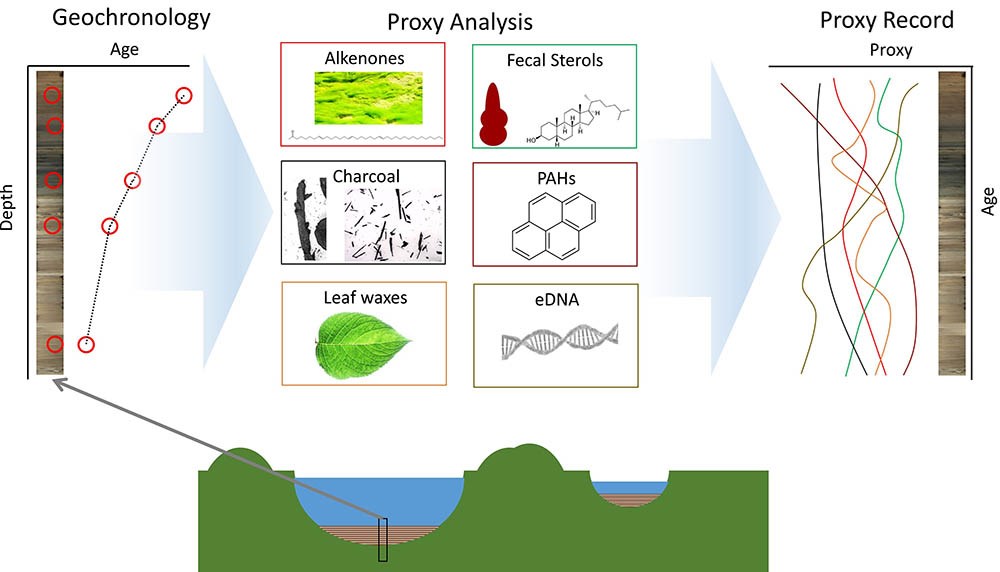
In this article, we highlight scientific tools and methods we are using to understand the climate and ecological history of Arctic Alaska. We have recently collected lake sediment cores from two lakes, Imuruk and Whitefish, in Bering Land Bridge National Preserve in northwest Alaska (Figure 3), to determine how temperature, plant ecology, and water chemistry have changed on the Seward Peninsula in the geologic past.
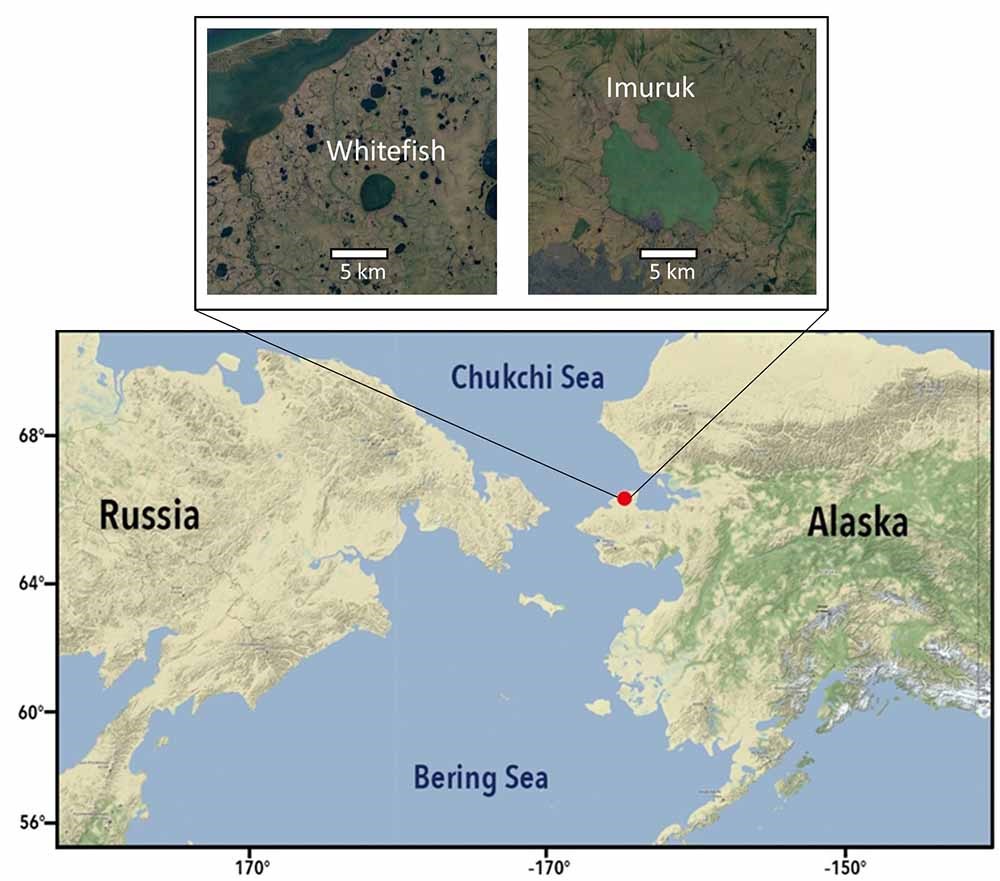
[Map adapted from Wang and others 2019.]
The lakes where we collected sediment cores are maars, meaning they formed when volcanic activity superheated ground ice or groundwater in permafrost terrain, building up pressure and eventually causing an explosion that formed the lake basin (Shackleton 1982, Begét et al. 1996). The exact ages of these lakes are debated, but recent evidence indicates that Imuruk may be more than 200,000 years old (Shackleton 1982, Burgess et al. 2019), meaning its sediments would offer the longest terrestrial sedimentary record of environmental change in Alaska from lakes and ocean sites (the ocean around the Bering Strait has no sediment during glacial times due the sea level drop and exposure). Imuruk may also have sediments older than most nearby marine records. To our knowledge, only one marine record, Integrated Ocean Drilling Program site U1343 on the Bering Sea shelf, contains sediments older than the last Glacial Maximum ca. 21,000 years ago (Westbrook 2014). However, even site U1343 sediments are limited to 150,000 years in age (Westbrook 2014), making Imuruk a truly exceptional archive. Our preliminary geochronological data from Whitefish Lake suggest it may have one of the highest sedimentation rates of all Alaskan lakes, which would offer one of the highest-resolution paleoenvironmental records in this region.
We hope that these sediment records can answer some very important questions about Alaska’s environmental history. These questions include: How has Alaska’s climate varied over time? How do fire regimes respond to climate variability? How do subsistence resources respond to climate changes? When did the first Americans arrive to Alaska and what was their impact on the environment?
How has Alaska’s climate varied over time?
There are several organic compounds preserved in lake sediments that can be used to reconstruct climates of the past. Alkenones are a class of organic molecules produced by certain algae living in these lakes (Wang et al. 2019). Alkenones are stable, or slow to degrade, and can be preserved for millennia in lake sediments. The relative amounts of different alkenones produced by these algae depend upon the water temperature of the lake during spring algal bloom (Longo et al. 2016). By measuring the relative concentrations of different alkenone molecules preserved in individual lake sediment horizons, we can reconstruct spring temperature changes.
Leaf waxes are another class of organic compounds preserved in lake sediments that can be measured to reconstruct past climatic conditions. Plants use leaf waxes to help conserve water and protect their tissues and their chemistry records the environmental conditions that the plant was exposed to (Eglinton and Hamilton 1967). Leaf wax compounds are composed of hydrogen and carbon atoms that originate from the water the plants take up, and the isotopic composition of water varies with climatic factors (e.g., wet versus dry time periods, temperature). By measuring the isotopic composition of plant leaf waxes preserved in lake sediments, we can reconstruct changes in climate (aridity) over time.
By reconstructing the climate, we can gain a better understanding of how climate in Alaska responds to external forcings, such as insolation or greenhouse gases, and we can assess the abnormality of rates of change for climatic changes like temperature and aridity. This is especially important in Alaska, where reliable instrumental records are far apart and brief relative to other places in the world. Using alkenones, we hope to extend the temperature record of this region past the earliest instrumental records. Additionally, by reconstructing climate, we can better understand ecosystem sensitives to changing climate by comparing our paleoclimate records with paleoecological data.
How do fire regimes respond to climate variability?
Wildfires produce numerous byproducts that can be preserved in lake sediments and used to reconstruct fire history. Charcoal particles produced by wildfires are transported to lake sediments by wind and water (Higuera et al. 2007, Vachula et al. 2018). By sieving lake sediments, we can isolate these tiny particles (greater than 125 microns in size; about the thickness of a human hair) and count them under a microscope. The changes in charcoal particle accumulation over time are a record of fire history. Like charcoal, polycyclic aromatic hydrocarbons (PAHs) are molecules that are produced by fire and preserved in lake sediment records (Argiriadis et al. 2018, Denis et al. 2012, Vachula et al. 2019). Total PAH abundance reflects fire activity and the relative amounts of different PAHs offer information about fire severity and the type of vegetation burned (conifers, deciduous plants, or moss; McGrath et al. 2003, Oros et al. 2006, Oros and Simoneit 2001a and 2001b,Yunker et al. 2002). In combination with the climate records reconstructed from alkenones and leaf waxes, these fire markers are used to infer how fire respond to climate.
Recent boreal forest and tundra fire seasons in Alaska have been particularly severe and are thought to be driven by increasing temperatures and aridity (Young et al. 2017). However, given the limited number of observational records, our understanding of fire-climate relationships could certainly be improved. For example, in 2007, the Anaktuvuk River Fire burned more than 250,000 acres (100,000 ha) of tundra on the North Slope of Alaska (Jones et al. 2009). At the time, it was thought that this fire was unprecedented (Hu et al. 2010, Higuera et al. 2011, Mack et al. 2011). But then researchers discovered that two similarly sized fires had burned on the supposedly fire-free North Slope between 1880 and 1920 (Jones et al. 2013). These new results suggest that fire activity may have been more common in the past than previously thought. It also suggests that the temperature sensitivity of tundra fires may be lower than what fire models predict (Higuera et al. 2009, Young et al. 2017). Fire frequency is generally higher in tundra regions of western Alaska (e.g., Bering Land Bridge National Preserve) than on the North Slope (e.g., northern reaches of Noatak National Preserve or Gates of the Arctic National Park and Preserve; (Racine et al. 1985, Sae-Lim et al. 2019), highlighting the need for regionally specific studies of fire-vegetation relationships (e.g., Racine et al. 2006). In this way, comparing tundra fire and climate reconstructions will be very helpful in understanding how fire activity, severity, and frequency respond to climate variability and change.
How do subsistence resources respond to climate changes?
Lakes provide crucial fish habitat, particularly for overwintering, rearing, and spawning. Anadromous fish species, such as salmon, are a critical component of both commercial and subsistence fishing throughout Alaska. Observation and modeling studies have predicted that as temperatures warm, subarctic fish may expand into Arctic waters (Fossheim et al. 2015, Wisz et al. 2015). However, the lack of long-term fish community records hinders our future conservation decision making because we have not observed this migration occur in recent decades. How did fish communities change on longer time scales in the Arctic before modern human intervention? Can we use past changes in the rate and extent of fish species migration as the basis to predict future changes and adapt to them accordingly?
Fortunately, similarly to alkenones, leaf waxes, and charcoal particles, DNA shed by fish and other organisms living in the lakes can also be preserved in sediment, even if there are no visible macrofossils present (Pansu et al. 2015). Those DNA fragments are called environmental DNA (eDNA) and are composed of DNA fragments from a variety of organisms living inside and nearby the lake. Recent advances in DNA sequencing techniques present unprecedented opportunities to identify the pre-sence of macroorganisms using eDNA in lake sediments. After eDNA is isolated from the sediment, it is purified, then amplified by polymerase chain reactions (PCR). During amplification, specific primers are used to target and replicate DNA fragments belonging to a particular species or other taxa of interest. Then, the DNA sequencer returns massive DNA sequence data from the amplicons and, by comparing those sequences to a DNA library, we can identify which species contributed to the eDNA over time and reconstruct the ecology of the lakes. Though there are uncertainties regarding ancient eDNA preservation, studies in upstate New York have successfully recovered yellow perch eDNA in 2,200 year-old lake sediment sample (Stager et al. 2015). It is possible that eDNA in Alaska lakes can provide a time series of fish community change as well as information on other organisms.
When did the first Americans arrive to Alaska and what was their impact on the environment?
Beringia, the ancient landmass that connected northeastern Asia and northwest North America during episodes of lower sea level, is thought to be the pathway by which humans arrived in the Americas (Goebel et al. 2008, Hoffecker et al. 2014). However, the timing of this human migration is hotly debated in archaeology and anthropology (Vachula et al. 2019, Waters 2019). The existing paradigm suggests that humans arrived in the Americas via the Bering Land Bridge in the late-Pleistocene (ca. 14,000 years ago; Goebel et al. 2008, Waters 2019). However, recent genetic research has suggested humans arrived in Beringia much earlier, during the height of the last ice age (ca. 30,000 years ago), and lived there for several thousand years before migrating south into the continents (Hoffecker et al. 2016, Tamm et al. 2007). Importantly, there is no archaeological evidence that unequivocally supports this genetically-inferred early human presence in Beringia (Hoffecker et al. 2020). At other lakes on the North Slope, we found sedimentary, albeit not archaeological, evidence of ice-age humans as early as 32,000 years ago in the form of fecal sterols, polycyclic aromatic hydrocarbons, and charcoal (Vachula et al. 2019). Fecal sterols are a class of molecules that are produced in animal feces and can be preserved in lake sediments (Argiriadis et al. 2018, D’Anjou et al. 2012). Animals, including humans, have unique fecal sterol signatures, so the composition of fecal sterols preserved in sediments can offer insight into the presence of humans in the past. By measuring fecal sterols preserved in sediments, we hope to determine when humans arrived to our study area and, subsequently with the paleofire records, what their environmental impact was over time.
Conclusion
Lake sediment cores from two lakes in Bering Land Bridge National Preserve in northwest Alaska are valuable environmental archives that can be used to better understand the geologic past of the Seward Peninsula. By analyzing proxies preserved in the sediments, such as alkenones, fecal sterols, charcoal, PAHs, leaf waxes, and eDNA, we can reconstruct how climate, ecology, and subsistence resources have varied through time as well as deduce the early environmental impacts of humans in this region. In light of the increasingly pronounced impacts of anthropogenic climate change in Arctic Alaska, a more thorough understanding of the past is the key to understanding what we see in the modern and what we can expect for the future.
Acknowledgements
Funding for this work was provided by the National Park Service’s Shared Beringia Heritage program, the Arctic Inventory and Monitoring Network, National Geographic Explorer program, and the Institute at Brown for Environment & Society. We thank Scott Amy of Skydance Aviation for providing aviation and logistical support.
References
Argiriadis, E., D. Battistel, D. B. McWethy, M. Vecchiato, T. Kirchgeorg, N. M. Kehrwald, C. Whitlock, J. M. Wilmshurst, and C. Barbante. 2018.
Lake sediment fecal and biomass burning biomarkers provide direct evidence for prehistoric human-lit fires in New Zealand. Scientific Reports 8: 12113.
Begét, J. E., D. M. Hopkins, and S. D. Charron. 1996.
The largest known maars on Earth, Seward Peninsula, northwest Alaska. Arctic 62–69.
Bekryaev, R. V., I. V. Polyakov, and V. A. Alexeev. 2010.
Role of polar amplification in long-term surface air temperature variations and modern Arctic warming. Journal of Climate 23: 3888–3906.
Binford, M. 1990.
Calculation and uncertainty analysis of 210Pb dates for PIRLA project lake sediment cores. Journal of Paleolimnology 3: 253–267.
Burgess, S. D., M. A. Coble, J. A. Vazquez, M. L. Coombs, and K. L. Wallace. 2019.
On the eruption age and provenance of the Old Crow tephra. Quaternary Science Reviews 207: 64–79.
D’Anjou, R. M., R. S. Bradley, N. L. Balascio, and D. B. Finkelstein. 2012.
Climate impacts on human settlement and agricultural activities in northern Norway revealed through sediment biogeochemistry. Proceedings of the National Academy of Sciences of the United States of America 109: 20332–7.
Denis, E. H., J. L. Toney, R. Tarozo, R. S. Anderson, L. D. Roach, and Y. Huang. 2012.
Polycyclic aromatic hydrocarbons (PAHs) in lake sediments record historic fire events: Validation using HPLC-fluorescence detection. Organic Geochemistry 45: 7–17.
Eglinton, G. and R. J. Hamilton. 1967.
Leaf epicuticular waxes. Science 156: 1322–1335.
Fossheim, M., R. Primicerio, E. Johannesen, R. B. Ingvaldsen, M. M. Aschan, and A. V. Dolgov. 2015.
Recent warming leads to a rapid borealization of fish communities in the Arctic. Nature Climate Change 5: 673–677.
Goebel, T., M. R. Waters, and D. H. O’Rourke. 2008.
The Late Pleistocene dispersal of modern humans in the Americas. Science 319: 1497-1502.
Gonzalez, P., F. Wang, M. Notaro, D. J. Vimont, and J. W. Williams. 2018.
Disproportionate magnitude of climate change in United States national parks. Environmental Research Letters 13: 104001.
Higuera, P. E., L. B. Brubaker, P. M. Anderson, F. S. Hu, and T. A. Brown. 2009.
Vegetation mediated the impacts of postglacial climate change on fire regimes in the south-central Brooks Range, Alaska. Ecological Monographs 79: 201–219.
Higuera, P. E., M. L. Chipman, J. L. Barnes, M. A. Urban, and F. S. Hu. 2011.
Variability of tundra fire regimes in Arctic Alaska: Millennial-scale patterns and ecological implications. Ecological Applications 21: 3211–3226.
Higuera, P. E., M. E. Peters, L. B. Brubaker, and D. G. Gavin. 2007.
Understanding the origin and analysis of sediment-charcoal records with a simulation model. Quaternary Science Reviews 26: 1790–1809.
Hoffecker, J. F., S. A. Elias, and D. H. O’Rourke. 2014.
Out of Beringia? Science 343(6174): 979-980.
Hoffecker, J. F., S. A. Elias, D. H. O’Rourke, G. R. Scott, and N. H. Bigelow. 2016.
Beringia and the global dispersal of modern humans. Evolutionary Anthropology: Issues, News, and Reviews 25: 64–78.
Hoffecker, J. F., S. A. Elias, and O. Potapova. 2020.
Arctic Beringia and Native American Origins. PaleoAmerica 6: 158–168.
Hu, F. S., P. E. Higuera, J. E. Walsh, W. L. Chapman, P. A. Duffy, L. B. Brubaker, and M. L. Chipman. 2010.
Tundra burning in Alaska: Linkages to climatic change and sea ice retreat. Journal of Geophysical Research 115: G04002.
Jones, B. M., A. L. Breen, B. V. Gaglioti, D. H. Mann, A. V. Rocha, G. Grosse, C. D. Arp, M. L. Kunz, and D. A. Walker. 2013.
Identification of unrecognized tundra fire events on the north slope of Alaska. Journal of Geophysical Research: Biogeosciences 118: 1334–1344.
Jones, B. M., C. A. Kolden, R. Jandt, J. T. Abatzoglou, F. Urban, and C. D. Arp. 2009.
Fire behavior, weather, and burn severity of the 2007 Anaktuvuk River tundra fire, North Slope, Alaska. Arctic, Antarctic, and Alpine Research 41: 309–316.
Longo, W. M., S. Theroux, A. E. Giblin, Y. Zheng, J. T. Dillon, and Y. Huang. 2016.
Temperature calibration and phylogenetically distinct distributions for freshwater alkenones: Evidence from northern Alaskan lakes. Geochimica et Cosmochimica Acta 180: 177–196.
Mack, M. C., M. S. Bret-Harte, T. N. Hollingsworth, R. R. Jandt, E. A. G. Schuur, G. R. Shaver, and D. L. Verbyla. 2011.
Carbon loss from an unprecedented Arctic tundra wildfire. Nature 475: 489–92.
McGrath, T. E., W. G.Chan, and M. R. Hajaligol. 2003.
Low temperature mechanism for the formation of polycyclic aromatic hydrocarbons from the pyrolysis of cellulose. Journal of Analytical and Applied Pyrolysis 66: 51–70.
Oros, D. R., M. R. Abas, N. Y. M. J. bin Omar, N. A. Rahman, and B. R. T. Simoneit. 2006.
Identification and emission factors of molecular tracers in organic aerosols from biomass burning: Part 3. Grasses. Applied Geochemistry 21: 919–940.
Oros, D. R. and B. R. T. Simoneit. 2001a.
Identification and emission factors of molecular tracers in organic aerosols from biomass burning Part 2. Deciduous trees. Applied Geochemistry 16: 1545–1565.
Oros, D. R. and B. R. T. Simoneit. 2001b.
Identification and emission factors of molecular tracers in organic aerosols from biomass burning Part 1. Temperate climate conifers. Applied Geochemistry 16: 1513–1544.
Pansu, J., C. Giguet-Covex, G. F. Ficetola, L. Gielly, F. Boyer, L. Zinger, F. Arnaud, J. Poulenard, P. Taberlet, and P. Choler. 2015.
Reconstructing long-term human impacts on plant communities: an ecological approach based on lake sediment DNA. Molecular Ecology 24: 1485–1498.
Racine, C., J. L. Allen, and J. G. Dennis. 2006.
Long-term monitoring of vegetation change following tundra fires in Noatak National Preserve, Alaska. Arctic Network of Parks Inventory and Monitoring Program, National Park Service, Alaska Region, Fairbanks, Alaska, USA.
Racine, C. H., J. G. Dennis, and W. A. Patterson, III. 1985.
Tundra fire regimes in the Noatak River watershed, Alaska: 1956-83. Arctic 38: 194–200.
Sae-Lim, J., J. M. Russell, R. S. Vachula, R. M. Holmes, P. J. Mann, J. D. Schade, and S. M. Natali. 2019.
Temperature-controlled tundra fire severity and frequency during the last millennium in the Yukon-Kuskokwim Delta, Alaska. Holocene 29(7): 095968361983803.
Serreze, M. C. and R. G. Barry. 2011.
Processes and impacts of Arctic amplification: A research synthesis. Global and Planetary Change 77: 85–96.
Shackleton, J. 1982.
Paleoenvironmental histories from whitefish and Imuruk lakes, Seward Peninsula, Alaska. Institute of Polar Studies, Ohio State University.
Stager, J. C., L. A. Sporn, M. Johnson, and S. Regalado. 2015.
Of paleo-genes and perch: What if an “Alien” as actually a native? PLoS ONE 10: e0119071.
Tamm, E., T. Kivisild, M. Reidla, M. Metspalu, D. G. Smith, C. J. Mulligan, C. M. Bravi, O. Rickards, C. Martinez-Labarga, E. K. Khusnutdinova, S. A. Fedorova, M. V. Golubenko, V. A. Stepanov, M. A. Gubina, S. I. Zhadanov, L. P. Ossipova, L. Damba, M. I. Voevoda, J. E. Dipierri, R. Villems, and R. S. Malhi. 2007.
Beringian Standstill and spread of Native American founders. PLoS ONE 2: e829.
Vachula, R. S., Y. Huang, W. M. Longo, S. G. Dee, W. C. Daniels, and J. M. Russell. 2019.
Evidence of Ice Age humans in eastern Beringia suggests early migration to North America. Quaternary Science Reviews 205: 35–44.
Vachula, R. S., J. M. Russell, Y. Huang, and N. Richter. 2018.
Assessing the spatial fidelity of sedimentary charcoal size fractions as fire history proxies with a high-resolution sediment record and historical data. Palaeogeography, Palaeoclimatology, Palaeoecology 508: 166–175.
Wang, K. J., J. A. O’Donnell, W. M. Longo, L. Amaral-Zettler, G. Li, Y. Yao, and Y. Huang. 2019.
Group I alkenones and Isochrysidales in the world’s largest maar lakes and their potential paleoclimate applications. Organic Geochemistry 138: 103924.
Waters, M .R. 2019.
Late Pleistocene exploration and settlement of the Americas by modern humans. Science 365: eaat5447.
Westbrook, R. E. 2014.
Evidence for a glacial refugium in south-central Beringia using modern analogs: A 152.2 kyr palynological record from IODP Expedition 323 sediment. M.S. thesis. University of Alaska, Fairbanks.
Wisz, M. S., O. Broennimann, P. Grønkjær, P. R. Møller, S. M. Olsen, D. Swingedouw, R. B. Hedeholm, E. E. Nielsen, A. Guisan, and L. Pellissier. 2015.
Arctic warming will promote Atlantic–Pacific fish interchange. Nature Climate Change 5: 261–265.
Young, A. M., P. E. Higuera, P. A. Duffy, and F. S. Hu. 2017.
Climatic thresholds shape northern high-latitude fire regimes and imply vulnerability to future climate change. Ecography 40: 606–617.
Yunker, M. B., R. W. Macdonald, R. Vingarzan, R. H. Mitchell, D. Goyette, and S. Sylvestre. 2002.
PAHs in the Fraser River basin: A critical appraisal of PAH ratios as indicators of PAH source and composition. Organic Geochemistry 33: 489–515.
Last updated: June 1, 2021